-
Evaluation of Differences in Solubilities in Organic Solvents of Wood-Based Industrial Kraft Lignins Using Hansen Parameters
-
Simple Green Purification of Spilanthol Derived from Natural Deep Eutectic Solvent and Ethanolic Acmella oleracea Extract
-
Volatile Compounds of Italian Honeys Assessed via HS-SPME-GC-MS for Authentication Using the Genetic Algorithm
Journal Description
Separations
Separations
- formerly Chromatography - is an international, peer-reviewed, open access journal on separation and purification science and technology in all areas of chemical, biological, physical science, and separation performance published monthly online by MDPI. The Central European Group of Separation Sciences (CEGSS) is affiliated with Separations and their members receive discounts on the article processing charges.
- Open Access— free for readers, with article processing charges (APC) paid by authors or their institutions.
- High Visibility: indexed within Scopus, SCIE (Web of Science), CAPlus / SciFinder, and other databases.
- Rapid Publication: manuscripts are peer-reviewed and a first decision is provided to authors approximately 15.1 days after submission; acceptance to publication is undertaken in 2.9 days (median values for papers published in this journal in the second half of 2024).
- Recognition of Reviewers: reviewers who provide timely, thorough peer-review reports receive vouchers entitling them to a discount on the APC of their next publication in any MDPI journal, in appreciation of the work done.
- Companion journal: Purification.
Impact Factor:
2.5 (2023);
5-Year Impact Factor:
2.6 (2023)
Latest Articles
Bioactive Properties of Pentacalia vaccinioides (Kunth) Cuatrec. (Asteraceae) Essential Oils: Evaluation of Antimicrobial and Antioxidant Activities
Separations 2025, 12(1), 9; https://doi.org/10.3390/separations12010009 - 5 Jan 2025
Abstract
Essential oils (EOs) have unique properties, such as antibacterial, antioxidant, and antiviral activities, which are beneficial in various industries, including cosmetics, food, and pharmaceuticals. In this study, the antioxidant and antimicrobial activities of Pentacalia vaccinioides EOs obtained from leaves and flowers (fresh and
[...] Read more.
Essential oils (EOs) have unique properties, such as antibacterial, antioxidant, and antiviral activities, which are beneficial in various industries, including cosmetics, food, and pharmaceuticals. In this study, the antioxidant and antimicrobial activities of Pentacalia vaccinioides EOs obtained from leaves and flowers (fresh and dried plant material) were evaluated using hydrodistillation (HD), steam distillation (SD), simultaneous distillation–extraction (SDE), and solid-phase microextraction (SPME) techniques. Antimicrobial activity (minimum inhibitory concentration, MIC) and antioxidant capacity (half-maximal inhibitory concentration, IC50) were determined. The identification and quantification of the compounds present in the EOs were conducted by gas chromatography coupled to mass spectrometry (GC-MS). The main secondary metabolites identified in most samples obtained by different extraction techniques included phenol (~18%), 1S-α-pinene (~15%), β-phellandrene (~13%), β-pinene (~12%), 4-terpineol (~10%), γ-terpinene (~10%), trans-nerolidol (~8%), limonene (~8%), and β-thujene (~6%). EOs obtained by HD, SD, and SDE exhibited antioxidant activity, with IC50 values between 621.7 and 696.6 µg/mL. Additionally, the EOs demonstrated bactericidal activity against Bacillus subtilis and Staphylococcus aureus, with MIC values of 5.0 and 45 µg/mL, respectively. Escherichia coli and Pseudomonas aeruginosa did not show antimicrobial susceptibility to EOs. This study constitutes the first evaluation of Pentacalia vaccinioides EOs, demonstrating their bioactive potential and the relevance of the extraction method. The findings highlight this species as a promising source of natural compounds for therapeutic and preservative applications, depending on the type of plant material and extraction technique used. Future research should investigate how microclimatic conditions and plant development affect the chemical composition and elucidate the molecular mechanisms behind the observed bioactivities to better understand their cellular actions. Furthermore, the evaluation of the applications of EOs and hydrolates in the pharmaceutical and food industries, along with the exploration of the bioactive potential of extraction-derived hydrolates, offers a promising avenue to maximize plant utility.
Full article
(This article belongs to the Special Issue Essential Oils: Extraction, Chemical Composition, and Bioactivities)
Open AccessArticle
Phytochemical Profile Screening and Selected Bioactivity of Myrtus communis Berries Extracts Obtained from Ultrasound-Assisted and Supercritical Fluid Extraction
by
Ilir Mërtiri, Gigi Coman, Mihaela Cotârlet, Mihaela Turturică, Nicoleta Balan, Gabriela Râpeanu, Nicoleta Stănciuc and Liliana Mihalcea
Separations 2025, 12(1), 8; https://doi.org/10.3390/separations12010008 - 3 Jan 2025
Abstract
This research paper investigates the phytochemical profile, antioxidant activity, antidiabetic potential, and antibacterial activity of Myrtus communis berries. Two extraction methods were employed to obtain the extracts: solid–liquid ultrasound-assisted extraction (UAE) and supercritical fluid extraction (SFE). The extracts were characterized using spectrophotometric methods
[...] Read more.
This research paper investigates the phytochemical profile, antioxidant activity, antidiabetic potential, and antibacterial activity of Myrtus communis berries. Two extraction methods were employed to obtain the extracts: solid–liquid ultrasound-assisted extraction (UAE) and supercritical fluid extraction (SFE). The extracts were characterized using spectrophotometric methods and Reversed-Phase High-Performance Liquid Chromatography (RP-HPLC). The UAE extract exhibited higher total flavonoid and anthocyanin content, while the SFE extract prevailed in total phenolic content and antioxidant activity in the DPPH radical screening assay. RP-HPLC characterization identified and quantified several polyphenolic compounds. In the UAE extract, epigallocatechin was found in a concentration of 2656.24 ± 28.15 µg/g dry weight (DW). In the SFE extract, cafestol was the identified compound with the highest content at a level of 29.65 ± 0.03 µg/g DW. Both extracts contained several anthocyanin compounds, including cyanidin 3-O-glucoside chloride, cyanidin-3-O-rutinoside chloride, malvidin-3-O-glucoside chloride, pelargonidin 3-O-glucoside chloride, peonidin 3-O-glucoside chloride, and peonidin-3-O-rutinoside chloride. The antidiabetic potential was evaluated in vitro by measuring the inhibition of α-amylase from porcine pancreas (type I-A). The results highlighted the ability of myrtle berry extracts to inhibit α-amylase enzymatic activity, suggesting its potential as an alternative for controlling postprandial hyperglycemia. The UAE extract showed the lowest IC50 value among the two extracts, with an average of 8.37 ± 0.52 µg/mL DW. The antibacterial activity of the extracts was assessed in vitro against Bacillus spp., Escherichia coli, and Staphylococcus aureus using the disk diffusion method. Both myrtle berry extracts exhibited similar antibacterial activity against the tested bacterial strains. The results support further investigation of myrtle berries extracts as a potential ingredient in functional food formulation, particularly due to its antioxidant, antidiabetic, and antibacterial properties.
Full article
(This article belongs to the Special Issue Green Extraction Techniques of Bioactive Compounds from Natural Sources)
►▼
Show Figures
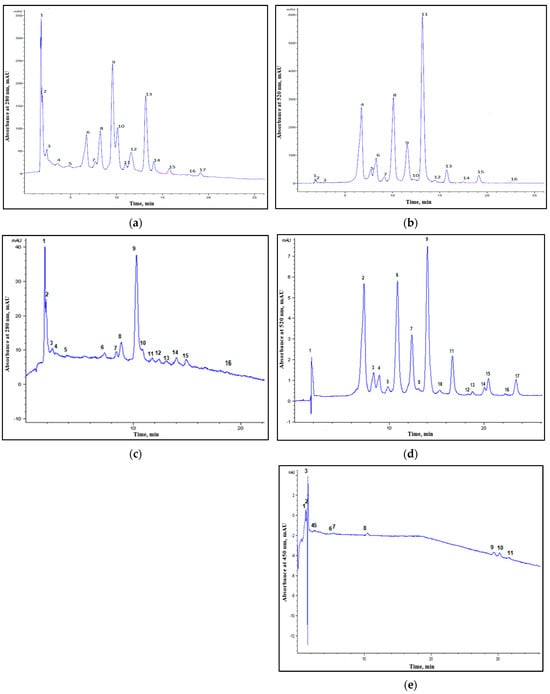
Figure 1
Figure 1
<p>Chromatograms of wild myrtle berries extracts. UAE extract: (<b>a</b>) 280 nm; 2—gallic acid; 5—epicatechin; 8—ferulic acid; 10—synapic acid; 1, 3, 4, 6, 7, 9, 11–17—unidentified compounds. (<b>b</b>) 520 nm; 2—gallic acid; 7—kuromanin chloride; 8—synapic acid; 10—naringin; 11—rutin trihydrate; 12—peonidin-3-<span class="html-italic">O</span>-rutinoside chloride; 15—quercetin; 1, 3–6, 9, 13, 14, 16—unidentified compounds. SFE extract: (<b>c</b>) 280 nm; 1—cafestol; 2—gallic acid; 7—ferulic acid; 16—quercetin; 3–6, 8–15—unidentified compounds. (<b>d</b>) 520 nm; 4—kuromanin chloride; 5—callistephin chloride; 9—oenin chloride; 1–3, 6–8, 10–17—unidentified compounds. (<b>e</b>) 450 nm: 8—zeaxanthin; 1–7, 9–11—unidentified compounds.</p> Full article ">Figure 2
<p>Antibacterial results of disk diffusion method against the tested bacterial strains. The samples codes represent the following: UAE—myrtle berries extract from ultrasound-assisted extraction; SFE—myrtle berries extract extract from supercritical fluid extraction; CC1—ciprofloxacin (positive control); CS—solubilization solvent (negative control).</p> Full article ">
<p>Chromatograms of wild myrtle berries extracts. UAE extract: (<b>a</b>) 280 nm; 2—gallic acid; 5—epicatechin; 8—ferulic acid; 10—synapic acid; 1, 3, 4, 6, 7, 9, 11–17—unidentified compounds. (<b>b</b>) 520 nm; 2—gallic acid; 7—kuromanin chloride; 8—synapic acid; 10—naringin; 11—rutin trihydrate; 12—peonidin-3-<span class="html-italic">O</span>-rutinoside chloride; 15—quercetin; 1, 3–6, 9, 13, 14, 16—unidentified compounds. SFE extract: (<b>c</b>) 280 nm; 1—cafestol; 2—gallic acid; 7—ferulic acid; 16—quercetin; 3–6, 8–15—unidentified compounds. (<b>d</b>) 520 nm; 4—kuromanin chloride; 5—callistephin chloride; 9—oenin chloride; 1–3, 6–8, 10–17—unidentified compounds. (<b>e</b>) 450 nm: 8—zeaxanthin; 1–7, 9–11—unidentified compounds.</p> Full article ">Figure 2
<p>Antibacterial results of disk diffusion method against the tested bacterial strains. The samples codes represent the following: UAE—myrtle berries extract from ultrasound-assisted extraction; SFE—myrtle berries extract extract from supercritical fluid extraction; CC1—ciprofloxacin (positive control); CS—solubilization solvent (negative control).</p> Full article ">
Open AccessArticle
Research on the Flotation Mechanism of Microemulsion Collector Enhanced Removal of Dyeing Impurities from Phosphogypsum
by
Xiaosheng Yu, Lijun Deng, Changpan Shen, Huiyong Li, Jingchao Li, Yijun Cao, Guoli Zhou and Guosheng Li
Separations 2025, 12(1), 7; https://doi.org/10.3390/separations12010007 - 31 Dec 2024
Abstract
Phosphogypsum is an industrial byproduct that is limited in its high-value application due to the presence of dyeing impurities (such as organic matter and carbon black). The flotation method has been verified to be effective in separating these dyeing impurities from gypsum. In
[...] Read more.
Phosphogypsum is an industrial byproduct that is limited in its high-value application due to the presence of dyeing impurities (such as organic matter and carbon black). The flotation method has been verified to be effective in separating these dyeing impurities from gypsum. In this study, microemulsion was used as the collector method of dyeing impurities for their separation from gypsum. The results of flotation tests showed that the microemulsion collector exhibited excellent collection capability and selectivity under natural pH conditions (pH = 1.5). With a microemulsion collector consumption of 400 g/t, purified gypsum of 65.1% whiteness, 95.74% yield, and 97.01% recovery was obtained. The purified gypsum of 65.1% whiteness, 95.74% yield, 97.01 recovery obtained by a used microemulsion collector amount of 400 g/t was better than using the same dosage of kerosene collector. The dispersion behavior of the microemulsion collector was studied by low-temperature transmission electron microscopy. The microemulsion collector demonstrated superior dispersibility, as it forms nano-oil droplets with an average size of 176.83 nm in the pulp, resolving issues associated with poor dispersibility observed in traditional kerosene collectors. Additionally, the nano-oil droplets effectively adsorbed onto the surface of dyeing impurities through hydrogen bonding, enhancing their hydrophobicity. Therefore, the microemulsion collector holds great potential for application in flotation whitening processes involving phosphogypsum.
Full article
(This article belongs to the Special Issue Separation and Extraction Technology in Mineral Processing)
►▼
Show Figures
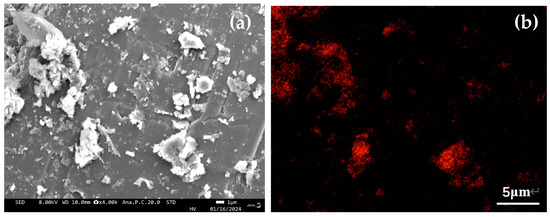
Figure 1
Figure 1
<p>SEM images of PG sample ((<b>a</b>) the microstructure of and (<b>b</b>) the elements mapping scanning of the PG raw ore.)</p> Full article ">Figure 2
<p>XRD pattern of the PG sample.</p> Full article ">Figure 3
<p>Flow chart of microemulsion preparation (<b>a</b>) and the reverse flotation tests (<b>b</b>).</p> Full article ">Figure 3 Cont.
<p>Flow chart of microemulsion preparation (<b>a</b>) and the reverse flotation tests (<b>b</b>).</p> Full article ">Figure 4
<p>TG-DTG pattern of the dyeing impurities.</p> Full article ">Figure 5
<p>Size distribution of oil droplets generated by the dispersion of the microemulsion (<b>a</b>) and kerosene (<b>b</b>).</p> Full article ">Figure 6
<p>Morphology of the microemulsion (<b>a</b>) and nano-oil droplets (<b>b</b>).</p> Full article ">Figure 7
<p>Flotation results at different dosages of kerosene (<b>a</b>) and microemulsion (<b>b</b>) collectors.</p> Full article ">Figure 8
<p>SEM images of raw PG (<b>a</b>) and gypsum concentrate (<b>b</b>).</p> Full article ">Figure 9
<p>Contact angles of gypsum (<b>a</b>) and dyeing impurities (<b>b</b>) before and after being treated with kerosene and microemulsion.</p> Full article ">Figure 10
<p>FT-IR results of gypsum and dyeing impurities treated by different collectors.</p> Full article ">Figure 11
<p>XPS results of dyeing impurities treated by different collectors.</p> Full article ">Figure 12
<p>Schematic diagram of the microemulsion collector enhancing flotation performance of PG.</p> Full article ">
<p>SEM images of PG sample ((<b>a</b>) the microstructure of and (<b>b</b>) the elements mapping scanning of the PG raw ore.)</p> Full article ">Figure 2
<p>XRD pattern of the PG sample.</p> Full article ">Figure 3
<p>Flow chart of microemulsion preparation (<b>a</b>) and the reverse flotation tests (<b>b</b>).</p> Full article ">Figure 3 Cont.
<p>Flow chart of microemulsion preparation (<b>a</b>) and the reverse flotation tests (<b>b</b>).</p> Full article ">Figure 4
<p>TG-DTG pattern of the dyeing impurities.</p> Full article ">Figure 5
<p>Size distribution of oil droplets generated by the dispersion of the microemulsion (<b>a</b>) and kerosene (<b>b</b>).</p> Full article ">Figure 6
<p>Morphology of the microemulsion (<b>a</b>) and nano-oil droplets (<b>b</b>).</p> Full article ">Figure 7
<p>Flotation results at different dosages of kerosene (<b>a</b>) and microemulsion (<b>b</b>) collectors.</p> Full article ">Figure 8
<p>SEM images of raw PG (<b>a</b>) and gypsum concentrate (<b>b</b>).</p> Full article ">Figure 9
<p>Contact angles of gypsum (<b>a</b>) and dyeing impurities (<b>b</b>) before and after being treated with kerosene and microemulsion.</p> Full article ">Figure 10
<p>FT-IR results of gypsum and dyeing impurities treated by different collectors.</p> Full article ">Figure 11
<p>XPS results of dyeing impurities treated by different collectors.</p> Full article ">Figure 12
<p>Schematic diagram of the microemulsion collector enhancing flotation performance of PG.</p> Full article ">
Open AccessArticle
Kinetics of Supercritical CO2 Extraction from Burrito (Aloysia polystachya) Leaves and Sucupira-Preta (Bowdichia virgilioides) Seeds
by
Gabrielle Vaz Vieira, Michel Rubens dos Reis Souza, Carlos Toshiyuki Hiranobe, José Eduardo Goncalves, Cristiane Mengue Feniman Moritz, Otávio Akira Sakai, Leila Maria Sotocorno e Silva, Michael Jones da Silva, Erivaldo Antônio da Silva, Renivaldo José dos Santos, Edson Antônio da Silva, Lucio Cardozo-Filho and Leandro Ferreira-Pinto
Separations 2025, 12(1), 6; https://doi.org/10.3390/separations12010006 - 31 Dec 2024
Abstract
►▼
Show Figures
This study investigated the application of supercritical carbon dioxide (CO2) for the extraction of essential oils from plant materials with anxiolytic potential, focusing on the leaves of burrito (Aloysia polystachya) and the seeds of sucupira-preta (Bowdichia virgilioides).
[...] Read more.
This study investigated the application of supercritical carbon dioxide (CO2) for the extraction of essential oils from plant materials with anxiolytic potential, focusing on the leaves of burrito (Aloysia polystachya) and the seeds of sucupira-preta (Bowdichia virgilioides). The supercritical extraction technique was chosen for its ability to produce high-purity extracts without residual solvents and to reduce the environmental impact. This study evaluated the influence of temperature (40 °C, 50 °C, and 60 °C) and pressure (22 MPa, 25 MPa, and 28 MPa) on extraction efficiency using a 22 factorial design with triplicates at the central point. The maximum yields were 1.2% for burrito leaves and 4.2% for sucupira-preta seeds. Despite their relatively low yields, the extracts contained a diverse range of chemical compounds, including fatty acids (oleic, linoleic, and palmitic acids), squalene, β-carotene, vitamin E, and other bioactive molecules with antioxidant, anti-inflammatory, and immunomodulatory properties. Statistical analysis demonstrated that pressure was the most influential factor affecting yield, whereas temperature played a secondary role. The Sovová kinetic model provided a good fit for the extraction curves, with determination coefficients (R2) above 0.95, thus validating the efficiency of the method. These results highlight the pharmaceutical potential of these extracts, particularly for therapeutic and anxiolytic purposes.
Full article
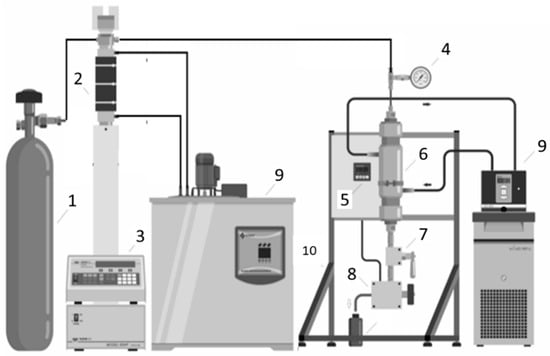
Figure 1
Figure 1
<p>A schematic of the experimental supercritical extraction unit: 1—CO<sub>2</sub> cylinder; 2—syringe pump; 3—thermostatic bath; 4—pressure indicator; 5—temperature controller/indicator; 6—extractor; 7—valve; 8—needle-type valve attached to an aluminum jacket for heating; 9—thermostatic bath; 10—aluminum structure.</p> Full article ">Figure 2
<p>Experimental kinetic extraction curves with supercritical CO<sub>2</sub> fitted using the Sovová model (<span class="html-fig-inline" id="separations-12-00006-i001"><img alt="Separations 12 00006 i001" src="/separations/separations-12-00006/article_deploy/html/images/separations-12-00006-i001.png"/></span>) of burrito leaves: 40 °C (■, 22 MPa; ▲, 28 MPa); 50 °C (<span class="html-fig-inline" id="separations-12-00006-i002"><img alt="Separations 12 00006 i002" src="/separations/separations-12-00006/article_deploy/html/images/separations-12-00006-i002.png"/></span>, 25 MPa); 60 °C (●, 22 MPa; ▼, 28 MPa) with a constant flow rate of 2.0 mL min<sup>−1</sup>.</p> Full article ">Figure 3
<p>Experimental kinetic extraction curves with supercritical CO<sub>2</sub> fitted using Sovová model (<span class="html-fig-inline" id="separations-12-00006-i001"><img alt="Separations 12 00006 i001" src="/separations/separations-12-00006/article_deploy/html/images/separations-12-00006-i001.png"/></span>) of sucupira-preta seeds: 40 °C (■, 22 MPa; ▲, 28 MPa); 50 °C (<span class="html-fig-inline" id="separations-12-00006-i002"><img alt="Separations 12 00006 i002" src="/separations/separations-12-00006/article_deploy/html/images/separations-12-00006-i002.png"/></span>, 25 MPa); 60 °C (●, 22 MPa; ▼, 28 MPa) with a constant flow rate of 2.0 mL min<sup>−1</sup>.</p> Full article ">Figure 4
<p>A response surface plot illustrating the extraction yield of oil from burrito leaves as a function of temperature and pressure, with a constant flow rate of 2.0 mL min<sup>−1</sup>.</p> Full article ">Figure 5
<p>A response surface plot showing the oil extraction yield from sucupira-preta seeds as influenced by temperature and pressure at a fixed flow rate of 2.0 mL min<sup>−1</sup>.</p> Full article ">Figure 6
<p>Pareto chart: analysis of linear effects of variables. (<b>A</b>) Burrito leaves and (<b>B</b>) sucupira-preta seeds.</p> Full article ">
<p>A schematic of the experimental supercritical extraction unit: 1—CO<sub>2</sub> cylinder; 2—syringe pump; 3—thermostatic bath; 4—pressure indicator; 5—temperature controller/indicator; 6—extractor; 7—valve; 8—needle-type valve attached to an aluminum jacket for heating; 9—thermostatic bath; 10—aluminum structure.</p> Full article ">Figure 2
<p>Experimental kinetic extraction curves with supercritical CO<sub>2</sub> fitted using the Sovová model (<span class="html-fig-inline" id="separations-12-00006-i001"><img alt="Separations 12 00006 i001" src="/separations/separations-12-00006/article_deploy/html/images/separations-12-00006-i001.png"/></span>) of burrito leaves: 40 °C (■, 22 MPa; ▲, 28 MPa); 50 °C (<span class="html-fig-inline" id="separations-12-00006-i002"><img alt="Separations 12 00006 i002" src="/separations/separations-12-00006/article_deploy/html/images/separations-12-00006-i002.png"/></span>, 25 MPa); 60 °C (●, 22 MPa; ▼, 28 MPa) with a constant flow rate of 2.0 mL min<sup>−1</sup>.</p> Full article ">Figure 3
<p>Experimental kinetic extraction curves with supercritical CO<sub>2</sub> fitted using Sovová model (<span class="html-fig-inline" id="separations-12-00006-i001"><img alt="Separations 12 00006 i001" src="/separations/separations-12-00006/article_deploy/html/images/separations-12-00006-i001.png"/></span>) of sucupira-preta seeds: 40 °C (■, 22 MPa; ▲, 28 MPa); 50 °C (<span class="html-fig-inline" id="separations-12-00006-i002"><img alt="Separations 12 00006 i002" src="/separations/separations-12-00006/article_deploy/html/images/separations-12-00006-i002.png"/></span>, 25 MPa); 60 °C (●, 22 MPa; ▼, 28 MPa) with a constant flow rate of 2.0 mL min<sup>−1</sup>.</p> Full article ">Figure 4
<p>A response surface plot illustrating the extraction yield of oil from burrito leaves as a function of temperature and pressure, with a constant flow rate of 2.0 mL min<sup>−1</sup>.</p> Full article ">Figure 5
<p>A response surface plot showing the oil extraction yield from sucupira-preta seeds as influenced by temperature and pressure at a fixed flow rate of 2.0 mL min<sup>−1</sup>.</p> Full article ">Figure 6
<p>Pareto chart: analysis of linear effects of variables. (<b>A</b>) Burrito leaves and (<b>B</b>) sucupira-preta seeds.</p> Full article ">
Open AccessArticle
A Compact Instrument for Temperature-Programming-Assisted Capillary–Nanoliquid Chromatography
by
Lincon Coutinho Marins, Alessandra Maffei Monteiro, Vivane Lopes Leal, Deyber Arley Vargas Medina, Edwin Martin Cardenas and Fernando Mauro Lanças
Separations 2025, 12(1), 5; https://doi.org/10.3390/separations12010005 - 30 Dec 2024
Abstract
The miniaturization of liquid chromatography (LC) columns to capillary and nanoscales allows temperature programming to be an effective alternative to solvent gradients for modulating eluotropic strength. This approach simplifies instrument design and operation, as a single pump can suffice to achieve efficient separations.
[...] Read more.
The miniaturization of liquid chromatography (LC) columns to capillary and nanoscales allows temperature programming to be an effective alternative to solvent gradients for modulating eluotropic strength. This approach simplifies instrument design and operation, as a single pump can suffice to achieve efficient separations. This study presents the development and application of a compact, lab-built high-pressure system for temperature-programmed capillary and nanoLC separations. The instrument includes a high-pressure capillary–nanoflow syringe pump, a time-based nanoliter injection system, a programmable capillary column oven for controlled temperature gradients, and a UV-Vis detection system with a custom nanoliter-scale detection cell. Each system component was designed and built in-house, with rigorous calibration to ensure accuracy and operational reliability. Experimental data confirm the system’s capability to deliver precise, reproducible temperature, and flow rates. Functionality was validated through temperature-programmed separations on packed and open tubular capillary columns. The results demonstrated that the developed instrument offers enhanced separation efficiency and reduced analysis time compared to isothermal methods, underscoring its potential for advanced applications in miniaturized liquid chromatography.
Full article
(This article belongs to the Special Issue Separation Techniques on a Miniaturized Scale)
►▼
Show Figures
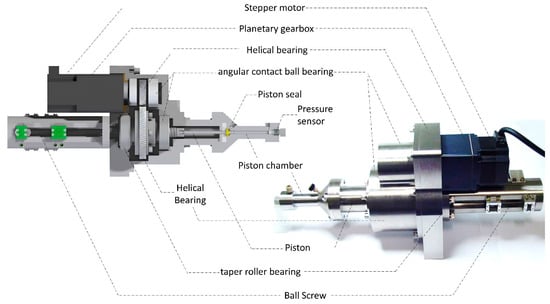
Figure 1
Figure 1
<p>Illustration of the mechanical architecture of the developed capillary–nanoflow high-pressure syringe pump, highlighting its main actuation components.</p> Full article ">Figure 2
<p>Photographs of the developed capillary–NanoLC temperature-programmable oven: (<b>a</b>) metal body of the oven; (<b>b</b>) Fiberfrax<sup>®</sup> thermal insulation fabric; (<b>c</b>) ceramic tube wrapped with resistive nickel–chromium heating wire; (<b>d</b>) cooling fan; (<b>e</b>) Celeron cooling duct; (<b>f</b>) servomotor controlling the cooling windows; (<b>g</b>) cooling windows; (<b>h</b>) fully assembled oven.</p> Full article ">Figure 3
<p>The assembly process of the capillary–NanoLC detection cell: (<b>a</b>) anodized aluminum blocks used for supporting the optical path length; (<b>b</b>) bending process of the capillary tube to form the optical path; (<b>c</b>) optical path length featuring 7.9 mm; (<b>d</b>) fully assembled detection cell, including the central metallic tube supporting the optical path length.</p> Full article ">Figure 4
<p>Measure vs. set flow rate: (<b>a</b>) in the 0–250 µL/min range; (<b>b</b>) in the 0–10 µL/min range; (<b>c</b>) in the 0–1.0 µL/min range.</p> Full article ">Figure 5
<p>Calibration of the injection volume: (<b>A</b>) a representative chromatogram of the mixture is used for calibration. The analysis used a fused silica capillary column (250 μm i.d., 15 cm length) packed with 3.0 μm C18 Pinnacle II (Restek) stationary phase. The mobile phase consisted of acetonitrile/water (48:62) acidified with 0.5% glacial acetic acid, delivered at a flow rate of 3 μL/min. Peak areas were calculated from chromatograms recorded at 240 nm; (<b>B</b>) relationship between the injection time and the injected volume, showing linearity in the 0–300 ms range and an exponential decrease in volume per unit time beyond this point; (<b>C</b>) correlation between the adjusted injection volume and the experimentally measured volume after calibration, demonstrating the accuracy of the system post-calibration.</p> Full article ">Figure 6
<p>Performance assessment of the developed temperature-programmable oven: (<b>a</b>) example of temperature control during an isothermal run at 60 °C; (<b>b</b>) correspondence between the temperature set by the user and the experimentally measured temperature; (<b>c</b>) relationship between the heating power required and the temperature ramp slope (°C/min); (<b>d</b>) an example of the oven’s operation during a run includes both isothermal and temperature gradient segments.</p> Full article ">Figure 7
<p>Chromatograms obtained using both the commercial microcell (Shimadzu) and the capillary cell developed in this work. The analysis was performed using a lab-made C-18 250 µm i.d ×150 mm packed column and a flow rate of 3.0 μL/min, with an injection volume of 60 nL. The elution order of the analytes was as follows: (1) naphthalene, (2) phenanthrene, and (3) anthracene. The concentration of each analyte was 30 mg/L.</p> Full article ">Figure 8
<p>Graphs showing variation as a function of the squared retention volume for the (<b>a</b>) lab-made and (<b>b</b>) commercial instruments.</p> Full article ">Figure 9
<p>Separation of PAHs (50 mg/mL) on a packed C18 capillary column (14 cm length, 250 µm i.d., 3.5 µm particle size) at a flow rate of 5.0 µL/min, using acetonitrile (70:30) as the mobile phase, under two conditions: (<b>a</b>) isothermal mode (no temperature programming) and (<b>b</b>) with temperature programming. Analytes: naphthalene (1), acenaphthylene (2), acenaphthene (3), fluorene (4), phenanthrene (5), anthracene (6), chrysene (7), and dibenzo(a,h)anthracene (8).</p> Full article ">Figure 10
<p>Separating alkylbenzene compounds using a WCOT column (5 m length, 50 μm i.d.): (<b>a</b>) without temperature programming and (<b>b</b>) with temperature programming. Peaks: uracil (1), Toluene (2), ethylbenzene (3), butylbenzene (4), pentylbenzene (5), and heptylbenzene (6).</p> Full article ">Figure 11
<p>Separating alkylbenzene compounds using a PLOT column (5 m length, 50 μm i.d.): (<b>a</b>) without temperature programming and (<b>b</b>) with temperature programming. Peaks: uracil (1), toluene (2), ethylbenzene (3), butylbenzene (4), pentylbenzene (5), and heptylbenzene (6).</p> Full article ">
<p>Illustration of the mechanical architecture of the developed capillary–nanoflow high-pressure syringe pump, highlighting its main actuation components.</p> Full article ">Figure 2
<p>Photographs of the developed capillary–NanoLC temperature-programmable oven: (<b>a</b>) metal body of the oven; (<b>b</b>) Fiberfrax<sup>®</sup> thermal insulation fabric; (<b>c</b>) ceramic tube wrapped with resistive nickel–chromium heating wire; (<b>d</b>) cooling fan; (<b>e</b>) Celeron cooling duct; (<b>f</b>) servomotor controlling the cooling windows; (<b>g</b>) cooling windows; (<b>h</b>) fully assembled oven.</p> Full article ">Figure 3
<p>The assembly process of the capillary–NanoLC detection cell: (<b>a</b>) anodized aluminum blocks used for supporting the optical path length; (<b>b</b>) bending process of the capillary tube to form the optical path; (<b>c</b>) optical path length featuring 7.9 mm; (<b>d</b>) fully assembled detection cell, including the central metallic tube supporting the optical path length.</p> Full article ">Figure 4
<p>Measure vs. set flow rate: (<b>a</b>) in the 0–250 µL/min range; (<b>b</b>) in the 0–10 µL/min range; (<b>c</b>) in the 0–1.0 µL/min range.</p> Full article ">Figure 5
<p>Calibration of the injection volume: (<b>A</b>) a representative chromatogram of the mixture is used for calibration. The analysis used a fused silica capillary column (250 μm i.d., 15 cm length) packed with 3.0 μm C18 Pinnacle II (Restek) stationary phase. The mobile phase consisted of acetonitrile/water (48:62) acidified with 0.5% glacial acetic acid, delivered at a flow rate of 3 μL/min. Peak areas were calculated from chromatograms recorded at 240 nm; (<b>B</b>) relationship between the injection time and the injected volume, showing linearity in the 0–300 ms range and an exponential decrease in volume per unit time beyond this point; (<b>C</b>) correlation between the adjusted injection volume and the experimentally measured volume after calibration, demonstrating the accuracy of the system post-calibration.</p> Full article ">Figure 6
<p>Performance assessment of the developed temperature-programmable oven: (<b>a</b>) example of temperature control during an isothermal run at 60 °C; (<b>b</b>) correspondence between the temperature set by the user and the experimentally measured temperature; (<b>c</b>) relationship between the heating power required and the temperature ramp slope (°C/min); (<b>d</b>) an example of the oven’s operation during a run includes both isothermal and temperature gradient segments.</p> Full article ">Figure 7
<p>Chromatograms obtained using both the commercial microcell (Shimadzu) and the capillary cell developed in this work. The analysis was performed using a lab-made C-18 250 µm i.d ×150 mm packed column and a flow rate of 3.0 μL/min, with an injection volume of 60 nL. The elution order of the analytes was as follows: (1) naphthalene, (2) phenanthrene, and (3) anthracene. The concentration of each analyte was 30 mg/L.</p> Full article ">Figure 8
<p>Graphs showing variation as a function of the squared retention volume for the (<b>a</b>) lab-made and (<b>b</b>) commercial instruments.</p> Full article ">Figure 9
<p>Separation of PAHs (50 mg/mL) on a packed C18 capillary column (14 cm length, 250 µm i.d., 3.5 µm particle size) at a flow rate of 5.0 µL/min, using acetonitrile (70:30) as the mobile phase, under two conditions: (<b>a</b>) isothermal mode (no temperature programming) and (<b>b</b>) with temperature programming. Analytes: naphthalene (1), acenaphthylene (2), acenaphthene (3), fluorene (4), phenanthrene (5), anthracene (6), chrysene (7), and dibenzo(a,h)anthracene (8).</p> Full article ">Figure 10
<p>Separating alkylbenzene compounds using a WCOT column (5 m length, 50 μm i.d.): (<b>a</b>) without temperature programming and (<b>b</b>) with temperature programming. Peaks: uracil (1), Toluene (2), ethylbenzene (3), butylbenzene (4), pentylbenzene (5), and heptylbenzene (6).</p> Full article ">Figure 11
<p>Separating alkylbenzene compounds using a PLOT column (5 m length, 50 μm i.d.): (<b>a</b>) without temperature programming and (<b>b</b>) with temperature programming. Peaks: uracil (1), toluene (2), ethylbenzene (3), butylbenzene (4), pentylbenzene (5), and heptylbenzene (6).</p> Full article ">
Open AccessReview
Advances in Recycling Technologies of Critical Metals and Resources from Cathodes and Anodes in Spent Lithium-Ion Batteries
by
Shuwen Wang, Yanrong Lai, Jingran Yang, Jiaxue Zhao, Yushan Zhang, Miaoling Chen, Jinfeng Tang, Junhua Xu and Minhua Su
Separations 2025, 12(1), 4; https://doi.org/10.3390/separations12010004 - 30 Dec 2024
Abstract
With the rapid economic development and the continuous growth in the demand for new energy vehicles and energy storage systems, a significant number of waste lithium-ion batteries are expected to enter the market in the future. Effectively managing the processing and recycling of
[...] Read more.
With the rapid economic development and the continuous growth in the demand for new energy vehicles and energy storage systems, a significant number of waste lithium-ion batteries are expected to enter the market in the future. Effectively managing the processing and recycling of these batteries to minimize environmental pollution is a major challenge currently facing the lithium-ion battery industry. This paper analyzes and compares the recycling strategies for different components of lithium-ion batteries, providing a summary of the main types of batteries, existing technologies at various pre-treatment stages, and recycling techniques for valuable resources such as heavy metals and graphite. Currently, pyrometallurgy and hydrometallurgy processes have matured; however, their high energy consumption and pollution levels conflict with the principles of the current green economy. As a result, innovative technologies have emerged, aiming to reduce energy consumption while achieving high recovery rates and minimizing the environmental impact. Nevertheless, most of these technologies are currently limited to the laboratory scale and are not yet suitable for large-scale application.
Full article
(This article belongs to the Section Purification Technology)
►▼
Show Figures
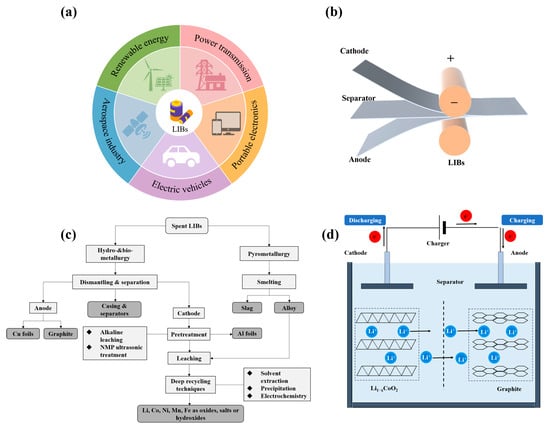
Figure 1
Figure 1
<p>(<b>a</b>) Applications of lithium-ion batteries; (<b>b</b>) The shape and components of some Li-ion battery configurations; (<b>c</b>) Flow-chart showing the typical recycling process; (<b>d</b>) Schematic diagram of the LIB working principle.</p> Full article ">Figure 2
<p>Schematic diagrams of pyrometallurgy, hydrometallurgy, and direct recovery processes [<a href="#B48-separations-12-00004" class="html-bibr">48</a>].</p> Full article ">Figure 3
<p>Characteristics of different pyrometallurgical technologies used to treat spent LIBs for the recovery of strategic metals.</p> Full article ">
<p>(<b>a</b>) Applications of lithium-ion batteries; (<b>b</b>) The shape and components of some Li-ion battery configurations; (<b>c</b>) Flow-chart showing the typical recycling process; (<b>d</b>) Schematic diagram of the LIB working principle.</p> Full article ">Figure 2
<p>Schematic diagrams of pyrometallurgy, hydrometallurgy, and direct recovery processes [<a href="#B48-separations-12-00004" class="html-bibr">48</a>].</p> Full article ">Figure 3
<p>Characteristics of different pyrometallurgical technologies used to treat spent LIBs for the recovery of strategic metals.</p> Full article ">
Open AccessArticle
CO2/CH4 and CO2/CO Selective Pebax-1657 Based Composite Hollow Fiber Membranes Prepared by a Novel Dip-Coating Technique
by
Dionysios S. Karousos, George V. Theodorakopoulos, Francesco Chiesa, Stéphan Barbe, Mirtat Bouroushian and Evangelos P. Favvas
Separations 2025, 12(1), 3; https://doi.org/10.3390/separations12010003 - 29 Dec 2024
Abstract
A novel and innovative method was developed to fabricate defect-free composite hollow fiber (HF) membranes using drop-casting under continuous flow. The synthesized Pebax-1657—based membranes were examined for gas separation processes, focusing on the separation of CO2 from CH4 and CO gases.
[...] Read more.
A novel and innovative method was developed to fabricate defect-free composite hollow fiber (HF) membranes using drop-casting under continuous flow. The synthesized Pebax-1657—based membranes were examined for gas separation processes, focusing on the separation of CO2 from CH4 and CO gases. The separation performance of the membranes was rigorously assessed under realistic binary gas mixture conditions to evaluate their selectivity and performance. The effect of pressure on separation performance was systematically investigated, with transmembrane pressures up to 10 bar being applied at a temperature of 298 K. Remarkable CO2/CH4 selectivities of up to 110 and CO2/CO selectivities of up to 48 were achieved, demonstrating the robustness and effectiveness of these composite HF membranes, suggesting their suitability for high-performance gas separation processes under varying operational conditions. Overall, this study introduces a novel approach for scaling up the fabrication of HF membranes and provides valuable insights into their application in CO2 separation technologies, offering the potential for advancements in areas such as natural gas processing and carbon capture from CO-containing streams.
Full article
(This article belongs to the Special Issue 10th Anniversary Special Issues: Membrane Separation Processes)
►▼
Show Figures
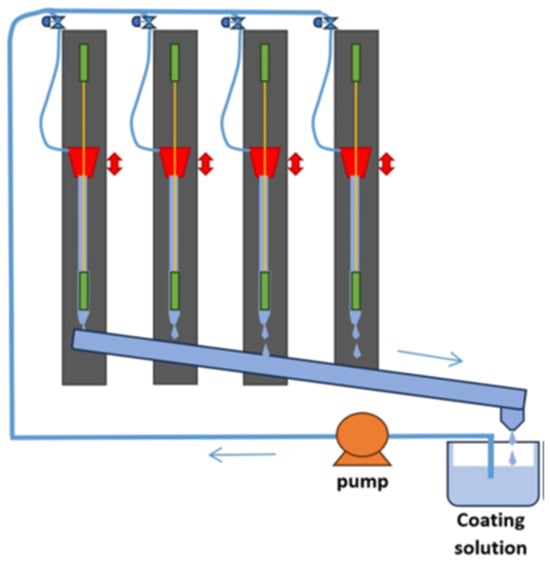
Figure 1
Figure 1
<p>Upscaled, according to a previous work [<a href="#B24-separations-12-00003" class="html-bibr">24</a>], HF coating device for underflow drop-casting.</p> Full article ">Figure 2
<p>SEM micrographs of the composite HF membrane: (<b>a</b>) cross-sectional view showing overall morphology; higher magnification images illustrating (<b>b</b>) the gutter layer; (<b>c</b>) the wall region with a sponge-like structure region; and (<b>d</b>) the separation layer thickness.</p> Full article ">Figure 3
<p>Separation factor (selectivity) and CO<sub>2</sub> permeance (GPU) as a function of pressure drop across the composite HF membrane for a gas mixture containing 10% <span class="html-italic">v</span>/<span class="html-italic">v</span> CO<sub>2</sub> in CH<sub>4</sub>. The temperature was maintained at 298 K.</p> Full article ">Figure 4
<p>Separation factor (selectivity) and CO<sub>2</sub> permeance (GPU) as a function of pressure drop across the composite HF membrane for a gas mixture containing 33% <span class="html-italic">v</span>/<span class="html-italic">v</span> CO<sub>2</sub> in CO. The temperature was maintained at 298 K.</p> Full article ">
<p>Upscaled, according to a previous work [<a href="#B24-separations-12-00003" class="html-bibr">24</a>], HF coating device for underflow drop-casting.</p> Full article ">Figure 2
<p>SEM micrographs of the composite HF membrane: (<b>a</b>) cross-sectional view showing overall morphology; higher magnification images illustrating (<b>b</b>) the gutter layer; (<b>c</b>) the wall region with a sponge-like structure region; and (<b>d</b>) the separation layer thickness.</p> Full article ">Figure 3
<p>Separation factor (selectivity) and CO<sub>2</sub> permeance (GPU) as a function of pressure drop across the composite HF membrane for a gas mixture containing 10% <span class="html-italic">v</span>/<span class="html-italic">v</span> CO<sub>2</sub> in CH<sub>4</sub>. The temperature was maintained at 298 K.</p> Full article ">Figure 4
<p>Separation factor (selectivity) and CO<sub>2</sub> permeance (GPU) as a function of pressure drop across the composite HF membrane for a gas mixture containing 33% <span class="html-italic">v</span>/<span class="html-italic">v</span> CO<sub>2</sub> in CO. The temperature was maintained at 298 K.</p> Full article ">
Open AccessArticle
Application of Magnetic Aquatic Plant Biochar for Efficient Removal of Antimony from Water: Adsorption Properties and Mechanism
by
Luyi Nan, Yuting Zhang, Min Liu, Liangyuan Zhao, Yuxuan Zhu and Xun Zhang
Separations 2025, 12(1), 2; https://doi.org/10.3390/separations12010002 - 28 Dec 2024
Abstract
Antimony (Sb) pollution in natural water bodies can cause significant harm to aquatic ecosystems. Currently, the utilization of chemicals in water bodies presents disadvantages, such as the hardship in collecting dispersed flocs and the incomplete elimination of pollutants. In the present research, a
[...] Read more.
Antimony (Sb) pollution in natural water bodies can cause significant harm to aquatic ecosystems. Currently, the utilization of chemicals in water bodies presents disadvantages, such as the hardship in collecting dispersed flocs and the incomplete elimination of pollutants. In the present research, a novel type of efficient adsorbent material for the magnetic recovery of Sb was proposed: the magnetic aquatic plant biochar. Its adsorption characteristics and mechanism were deeply investigated. The results demonstrated that, among the three types of aquatic plants, the magnetic biochar of Arundo donax magnetic biochar (LMBC) displayed the most superior adsorption effect on Sb. Under optimal adsorption conditions (pyrolysis temperature of 300 °C, dosage of 100 mg, pH of 8), the removal rate of Sb by LMBC exceeded 97%. The adsorption rate of Sb by LMBC was relatively rapid, and the kinetics of adsorption conformed to a pseudo-second-order kinetic model. The adsorption isotherm was consistent with the Langmuir and Freundlich models, and the maximum adsorption capacity of Sb reached 26.07 mg/g, suggesting that the adsorption process pertained to the adsorption of multi-molecular layers. The influence of coexisting ions on the adsorption effect of LMBC was insignificant. The SEM characterization results revealed that LMBC mainly consisted of the elements C and O. The BET characterization results demonstrated that the magnetization modification augmented the specific surface area by approximately 30 times to reach 89.14 m2/g, and the pore volume increased by twofold to 0.18 cm3/g, creating a favorable condition for Sb adsorption. The FTIR, XRD, and XPS results indicated that the surface of LMBC was rich in carboxyl and hydroxyl groups and was successfully loaded with Fe2O3 and Fe3O4. LMBC not only facilitates the resourceful utilization of aquatic plant waste but also effectively removes antimony (Sb) pollution through its magnetic properties. This dual functionality presents promising application prospects for the efficient adsorption and removal of Sb from water.
Full article
(This article belongs to the Special Issue Adsorption of Emerging Water Pollutants by Advanced Materials)
►▼
Show Figures
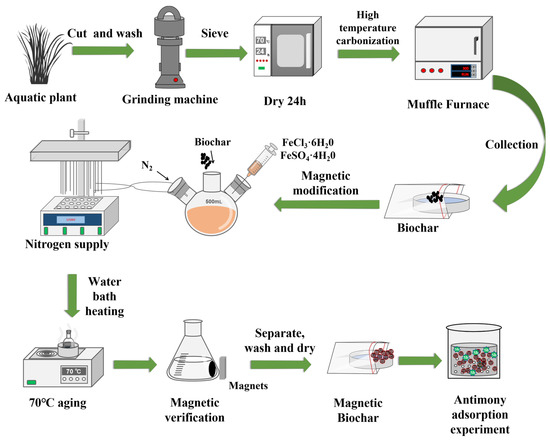
Figure 1
Figure 1
<p>The preparation process of the biochars and magnetic biochars.</p> Full article ">Figure 2
<p>Adsorption and desorption isothermal curves of LBC (<b>a</b>) and LMBC (<b>b</b>).</p> Full article ">Figure 3
<p>Scanning electron micrographs of LBC and LMBC before and after adsorption. (<b>a</b>) LBC-before adsorption; (<b>b</b>) LBC-after adsorption; (<b>c</b>) LMBC-before adsorption; (<b>d</b>) LMBC-after adsorption.</p> Full article ">Figure 4
<p>C, N, O, and Fe energy spectrum fluorescence of LBC and LMBC.</p> Full article ">Figure 5
<p>The FTlR results of LBC and LMBC before and after absorption.</p> Full article ">Figure 6
<p>XRD pattern of LBC and LMBC before and after adsorption.</p> Full article ">Figure 7
<p>XPS spectra of LMBC before and after adsorption. (<b>a</b>) Full spectrum; (<b>b</b>) C1s fine spectrum; (<b>c</b>) O1s fine spectrum; (<b>d</b>) Fe2p fine spectrum; and (<b>e</b>) Sb3d fine spectrum.</p> Full article ">Figure 7 Cont.
<p>XPS spectra of LMBC before and after adsorption. (<b>a</b>) Full spectrum; (<b>b</b>) C1s fine spectrum; (<b>c</b>) O1s fine spectrum; (<b>d</b>) Fe2p fine spectrum; and (<b>e</b>) Sb3d fine spectrum.</p> Full article ">Figure 8
<p>Magnetization curve of LMBC.</p> Full article ">Figure 9
<p>(<b>a</b>) Effect of biochar and magnetic biochar prepared by different aquatic plant species; (<b>b</b>) effect of biochar and magnetic biochar dosage; (<b>c</b>) effect of initial pH; and (<b>d</b>) effect of ionic strength. “****”: the number of asterisks denotes the level of statistical significance, with four asterisks indicating a <span class="html-italic">p</span>-value of less than 0.0001, signifying a highly significant difference between LBC and LMBC.</p> Full article ">Figure 10
<p>(<b>a</b>) Adsorption kinetics of LBC; (<b>b</b>) adsorption kinetics of LMBC; (<b>c</b>,<b>d</b>) adsorption isotherm of LBC; and (<b>e</b>,<b>f</b>) adsorption isotherm of LMBC (experimental conditions: 150 mL of 5 mg/L Sb solution with 100 mg LMBC at the initial pH of 8).</p> Full article ">Figure 11
<p>Schematic diagram of the mechanism of Sb adsorption and removal by LMBC.</p> Full article ">
<p>The preparation process of the biochars and magnetic biochars.</p> Full article ">Figure 2
<p>Adsorption and desorption isothermal curves of LBC (<b>a</b>) and LMBC (<b>b</b>).</p> Full article ">Figure 3
<p>Scanning electron micrographs of LBC and LMBC before and after adsorption. (<b>a</b>) LBC-before adsorption; (<b>b</b>) LBC-after adsorption; (<b>c</b>) LMBC-before adsorption; (<b>d</b>) LMBC-after adsorption.</p> Full article ">Figure 4
<p>C, N, O, and Fe energy spectrum fluorescence of LBC and LMBC.</p> Full article ">Figure 5
<p>The FTlR results of LBC and LMBC before and after absorption.</p> Full article ">Figure 6
<p>XRD pattern of LBC and LMBC before and after adsorption.</p> Full article ">Figure 7
<p>XPS spectra of LMBC before and after adsorption. (<b>a</b>) Full spectrum; (<b>b</b>) C1s fine spectrum; (<b>c</b>) O1s fine spectrum; (<b>d</b>) Fe2p fine spectrum; and (<b>e</b>) Sb3d fine spectrum.</p> Full article ">Figure 7 Cont.
<p>XPS spectra of LMBC before and after adsorption. (<b>a</b>) Full spectrum; (<b>b</b>) C1s fine spectrum; (<b>c</b>) O1s fine spectrum; (<b>d</b>) Fe2p fine spectrum; and (<b>e</b>) Sb3d fine spectrum.</p> Full article ">Figure 8
<p>Magnetization curve of LMBC.</p> Full article ">Figure 9
<p>(<b>a</b>) Effect of biochar and magnetic biochar prepared by different aquatic plant species; (<b>b</b>) effect of biochar and magnetic biochar dosage; (<b>c</b>) effect of initial pH; and (<b>d</b>) effect of ionic strength. “****”: the number of asterisks denotes the level of statistical significance, with four asterisks indicating a <span class="html-italic">p</span>-value of less than 0.0001, signifying a highly significant difference between LBC and LMBC.</p> Full article ">Figure 10
<p>(<b>a</b>) Adsorption kinetics of LBC; (<b>b</b>) adsorption kinetics of LMBC; (<b>c</b>,<b>d</b>) adsorption isotherm of LBC; and (<b>e</b>,<b>f</b>) adsorption isotherm of LMBC (experimental conditions: 150 mL of 5 mg/L Sb solution with 100 mg LMBC at the initial pH of 8).</p> Full article ">Figure 11
<p>Schematic diagram of the mechanism of Sb adsorption and removal by LMBC.</p> Full article ">
Open AccessArticle
Irradiated Gao Miao Zi Bentonite for Uranium Retention: Performance and Mechanism
by
Yushan Zhang, Gang Song, Yujie Mo, Shuwen Wang, Diyun Chen and Minhua Su
Separations 2025, 12(1), 1; https://doi.org/10.3390/separations12010001 - 26 Dec 2024
Abstract
Bentonite has been considered as backfill material in the long-term deep geological disposal sites for radioactive waste. The performance of raw and irradiated bentonite based on the retention of radioactive nuclides, such as U(VI), is a critical factor for its application. Herein, the
[...] Read more.
Bentonite has been considered as backfill material in the long-term deep geological disposal sites for radioactive waste. The performance of raw and irradiated bentonite based on the retention of radioactive nuclides, such as U(VI), is a critical factor for its application. Herein, the intrinsic features and adsorption behavior of Gao Miao Zi (GMZ) bentonite based on uranyl ions was investigated. In aqueous solutions, bentonite can achieve an adsorption rate of up to 100% for U(VI). The primary mechanism of U(VI) adsorption by GMZ bentonite is ion exchange, supplemented by surface complexation. Strong irradiation can introduce slight structural changes and framework fractures in bentonite, reducing its adsorption capacity for U(VI). This study provides an in-depth analysis of the adverse effects of high doses of radiation (100 kGy) on the microstructure and adsorption properties of bentonite, offering important insights for the safe storage of radioactive waste.
Full article
(This article belongs to the Special Issue Separation Technology for Metal Extraction and Removal)
►▼
Show Figures
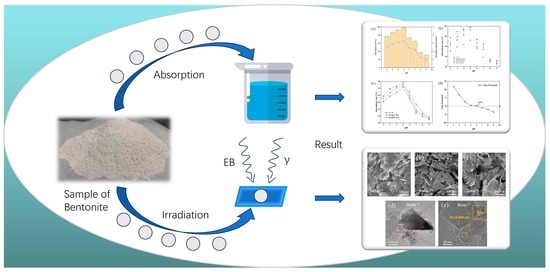
Graphical abstract
Graphical abstract
Full article ">Figure 1
<p>SEM images of (<b>a</b>) natural GMZ bentonite, (<b>b</b>) electron-beam-irradiated bentonite, and (<b>c</b>) gamma-irradiated bentonite, as well as (<b>d</b>) TEM image of natural GMZ bentonite and (<b>e</b>) TEM high-resolution image.</p> Full article ">Figure 2
<p>(<b>a</b>) The SEM elemental mapping images, (<b>b</b>) elemental composition, and (<b>c</b>) elemental content of the GMZ bentonite.</p> Full article ">Figure 3
<p>(<b>a</b>) XRD pattern, (<b>b</b>) FT-IR spectra, (<b>c</b>) N<sub>2</sub> adsorption-desorption isotherm, and (<b>d</b>) pore size distribution curve of GMZ bentonite before and after irradiation.</p> Full article ">Figure 4
<p>(<b>a</b>) Effect of the contact time and dosing amount on the uranium adsorption rate based on the adsorption of GMZ bentonite (uranium initial = 20 mg/L, pH = 5, T = room temperature); (<b>b</b>) effect of the initial uranium concentration on the absorption rate and adsorption capacity based on the adsorption of GMZ bentonite (dosage = 6 g/L, pH = 5, t = 30 min, and T = room temperature).</p> Full article ">Figure 5
<p>Effect of (<b>a</b>) pH (uranium initial = 20 mg/L, T = room temperature, m/V = 6 g/L); (<b>b</b>) ionic strength (uranium initial = 20 mg/L, T = room temperature, m/V = 6 g/L); (<b>c</b>) HA concentration (uranium initial = 20 mg/L, T = room temperature, m/V = 6 g/L, I = 0.1 M NaCl); (<b>d</b>) zeta potentials of bentonite as a function of pH (uranium initial = 20 mg/L, T = room temperature, m/V = 6 g/L).</p> Full article ">Figure 6
<p>(<b>a</b>) Kinetic model; (<b>b</b>) particle diffusion model; (<b>c</b>) isothermal model of GMZ bentonite for the adsorption of uranium.</p> Full article ">Figure 7
<p>(<b>a</b>) TEM, (<b>b</b>) TEM-EDS, (<b>c</b>) XRD, (<b>d</b>) FT-IR, and (<b>e</b>) XPS full-survey spectra, as well as the (<b>f</b>) U 4f XPS spectra of the GMZ bentonite before and after the absorption of U(VI).</p> Full article ">Figure 8
<p>The possible mechanism of uranium adsorption by GMZ bentonite.</p> Full article ">
Full article ">Figure 1
<p>SEM images of (<b>a</b>) natural GMZ bentonite, (<b>b</b>) electron-beam-irradiated bentonite, and (<b>c</b>) gamma-irradiated bentonite, as well as (<b>d</b>) TEM image of natural GMZ bentonite and (<b>e</b>) TEM high-resolution image.</p> Full article ">Figure 2
<p>(<b>a</b>) The SEM elemental mapping images, (<b>b</b>) elemental composition, and (<b>c</b>) elemental content of the GMZ bentonite.</p> Full article ">Figure 3
<p>(<b>a</b>) XRD pattern, (<b>b</b>) FT-IR spectra, (<b>c</b>) N<sub>2</sub> adsorption-desorption isotherm, and (<b>d</b>) pore size distribution curve of GMZ bentonite before and after irradiation.</p> Full article ">Figure 4
<p>(<b>a</b>) Effect of the contact time and dosing amount on the uranium adsorption rate based on the adsorption of GMZ bentonite (uranium initial = 20 mg/L, pH = 5, T = room temperature); (<b>b</b>) effect of the initial uranium concentration on the absorption rate and adsorption capacity based on the adsorption of GMZ bentonite (dosage = 6 g/L, pH = 5, t = 30 min, and T = room temperature).</p> Full article ">Figure 5
<p>Effect of (<b>a</b>) pH (uranium initial = 20 mg/L, T = room temperature, m/V = 6 g/L); (<b>b</b>) ionic strength (uranium initial = 20 mg/L, T = room temperature, m/V = 6 g/L); (<b>c</b>) HA concentration (uranium initial = 20 mg/L, T = room temperature, m/V = 6 g/L, I = 0.1 M NaCl); (<b>d</b>) zeta potentials of bentonite as a function of pH (uranium initial = 20 mg/L, T = room temperature, m/V = 6 g/L).</p> Full article ">Figure 6
<p>(<b>a</b>) Kinetic model; (<b>b</b>) particle diffusion model; (<b>c</b>) isothermal model of GMZ bentonite for the adsorption of uranium.</p> Full article ">Figure 7
<p>(<b>a</b>) TEM, (<b>b</b>) TEM-EDS, (<b>c</b>) XRD, (<b>d</b>) FT-IR, and (<b>e</b>) XPS full-survey spectra, as well as the (<b>f</b>) U 4f XPS spectra of the GMZ bentonite before and after the absorption of U(VI).</p> Full article ">Figure 8
<p>The possible mechanism of uranium adsorption by GMZ bentonite.</p> Full article ">
Open AccessArticle
The Effect of Plasma Pretreatment on the Flotation of Lithium Aluminate and Gehlenite Using Light-Switchable Collectors
by
Ali Zgheib, Maximilian Hans Fischer, Stéphanie Mireille Tsanang, Iliass El Hraoui, Shukang Zhang, Annett Wollmann, Alfred P. Weber, Ursula E. A. Fittschen, Thomas Schirmer and Andreas Schmidt
Separations 2024, 11(12), 362; https://doi.org/10.3390/separations11120362 - 23 Dec 2024
Abstract
The pyridinium phenolate punicine is a switchable molecule from Punica granatum. Depending on the pH, punicine exists as a cation, neutral molecule, anion, or dianion. In addition, punicine reacts to light, under the influence of which it forms radical species. We report
[...] Read more.
The pyridinium phenolate punicine is a switchable molecule from Punica granatum. Depending on the pH, punicine exists as a cation, neutral molecule, anion, or dianion. In addition, punicine reacts to light, under the influence of which it forms radical species. We report on three punicine derivatives that possess an adamantyl, 2-methylnonyl, or heptadecyl substituent and on their performance in the flotation of lithium aluminate, an engineered artificial mineral (EnAM) for the recycling of lithium, e.g., from lithium-ion batteries. By optimizing the parameters: pH and light conditions (daylight, darkness), recovery rates of 92% of LiAlO2 are achieved. In all cases, the flotation of the gangue material gehlenite (Ca2Al[AlSiO7]) is suppressed. IR, the contact angle, zeta potential measurements, TG-MS, and PXRD confirm that the punicines interact with the surface of LiAlO2, which is covered by LiAl2(OH)7 after contact to water, resulting in a hydrophobization of the particle. The plasma pretreatment of the lithium aluminate has a significant influence on the flotation results and increases the recovery rates of lithium aluminate in blank tests by 58%. The oxidative plasma leads to a partial dehydratisation of the LiAl2(OH)7 and thus to a hydrophobization of the particles, while a reductive plasma causes a more hydrophilic particle surface.
Full article
(This article belongs to the Special Issue Green Separation and Purification Technology)
►▼
Show Figures
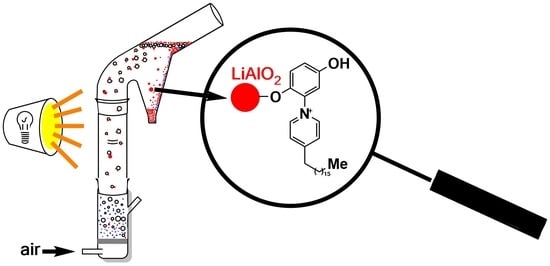
Graphical abstract
Graphical abstract
Full article ">Figure 1
<p>TGA measurements of pure lithium aluminate and 5 min oxidative plasma-pretreated lithium aluminate samples.</p> Full article ">Figure 2
<p>TG-MS of pure LiAlO<sub>2</sub> (<b>left</b>) and 5 min plasma pretreated LiAlO<sub>2</sub> (<b>right</b>).</p> Full article ">Figure 3
<p>Comparison of the recovery rates of lithium aluminate at different light scenarios in the presence of the collectors <b>1</b>–<b>3</b> under identical conditions, i.e., 2.00 g of LiAlO<sub>2</sub>, 1 min of mixing (500 rpm) with 25 mL of distilled water, 60 µL (1 × 10<sup>−5</sup> M) of the corresponding collector, 1 min of mixing, 30 µL of frother, and another 1 min of mixing. The flotation was done for 3 min at an air flow rate of 32 mL/min using 250 mL distilled water in daylight at a pH of 10.9 ± 0.3.</p> Full article ">Figure 4
<p>Comparison of the recovery rates of lithium aluminate at different pH values in the presence of the collectors <b>1</b>–<b>3</b> under identical conditions, i.e., 2.00 g of LiAlO<sub>2</sub>, 1 min of mixing (500 rpm) with 25 mL of distilled water, 60 µL (1 × 10<sup>−5</sup> M) of the corresponding collector, 1 min of mixing, 30 µL of frother, and another 1 min of mixing. The flotation was done for 3 min at an air flow rate of 32 mL/min using 250 mL distilled water in daylight.</p> Full article ">Figure 5
<p>Single mineral flotation of lithium aluminate and gehlenite using punicine <b>3</b> as collector at pH 10.9 ± 0.3 for lithium aluminate and pH 8.3 ± 0.3 for gehlenite at different light scenarios. The conditioning was as follows: 120 µL collector, 1 min mixing, 30 µL frother, 1 min mixing, stirring speed 500 rpm, air flow rate of 32 cm<sup>3</sup>/min using 250 mL distilled water, and flotation time of 3 min.</p> Full article ">Figure 6
<p>Single mineral flotation of plasma-pretreated lithium aluminate and the gehlenite-rich material without collector at pH 10.9 ± 0.3 for lithium aluminate and pH 8.3 ± 0.3 for gehlenite as a function of plasma treatment time. Conditioning was as follows: 30 µL frother, 1 min mixing, stirring speed 500 rpm, air flow rate of 32 cm<sup>3</sup>/min using 250 mL distilled water, and flotation time of 3 min.</p> Full article ">Figure 7
<p>Single mineral flotation of the oxidative plasma pretreated lithium aluminate and gehlenite using punicine <b>3</b> as a collector at pH 10.9 ± 0.3 for lithium aluminate, and pH 8.3 for gehlenite as a function of plasma treatment time in min. The conditioning was as follows: 60 µL collector, 1 min mixing 30 µL frother, 1 min mixing, stirring speed of 500 rpm, air flow rate of 32 cm<sup>3</sup>/min using 250 mL distilled water, and applying a flotation time of 3 min.</p> Full article ">Figure 8
<p>Single mineral flotation of the reductive plasma pretreated lithium aluminate in blank and using punicine <b>3</b> as a collector at pH 10.9 ± 0.3 as a function of plasma treatment time in min. The conditioning was as follows: 60 µL collector, 1 min mixing 30 µL frother, 1 min mixing, stirring speed of 500 rpm, air flow rate of 32 cm<sup>3</sup>/min using 250 mL distilled water, and flotation time of 3 min.</p> Full article ">Figure 9
<p>Contact angle on LiAlO<sub>2</sub> before and after flotation with punicine <b>3</b> under identical conditions, i.e., 2.00 g of the mineral, 1 min mixing (500 rpm) with 25 mL distilled water, 120 µL (2 × 10<sup>−5</sup> M) of the corresponding collector, 1 min mixing, 30 µL foaming agent, and 1 min mixing again. Flotation was carried out for 3 min at an air flow rate of 32 mL/min with 250 mL of distilled water in daylight and a pH of 10.9 ± 0.3.</p> Full article ">Figure 10
<p>Zeta potential of pure lithium aluminate, lithium aluminate with 7.2 µL of 42.5 mmol/L punicine <b>1</b>, lithium aluminate with 7.2 µL of 42.5 mmol/L punicine <b>2,</b> and lithium aluminate with 7.2 µL of 42.5 mmol/L punicine <b>3</b> in distilled water and under different pH values.</p> Full article ">Figure 11
<p>FTIR spectra of LiAlO<sub>2</sub> in the presence of 60 µL 42.5 µmol/L punicine <b>3</b> with and without pretreatment with plasma.</p> Full article ">Figure 12
<p>Zeta potential of pure and oxidative plasma-pretreated lithium aluminate, with and without 10 µL of 42.5 mmol/L punicine <b>3</b> at pH 10.9 ± 0.3.</p> Full article ">Scheme 1
<p>Schematic representation of the switchability of punicine with respect to charges and radical status.</p> Full article ">Scheme 2
<p>Intermolecular interactions and complexes of punicine.</p> Full article ">Scheme 3
<p>Synthesis of the punicine derivatives.</p> Full article ">Scheme 4
<p>Reactions of lithium aluminate surfaces with water.</p> Full article ">Scheme 5
<p>Interactions of punicines with lithium aluminum surfaces.</p> Full article ">
Full article ">Figure 1
<p>TGA measurements of pure lithium aluminate and 5 min oxidative plasma-pretreated lithium aluminate samples.</p> Full article ">Figure 2
<p>TG-MS of pure LiAlO<sub>2</sub> (<b>left</b>) and 5 min plasma pretreated LiAlO<sub>2</sub> (<b>right</b>).</p> Full article ">Figure 3
<p>Comparison of the recovery rates of lithium aluminate at different light scenarios in the presence of the collectors <b>1</b>–<b>3</b> under identical conditions, i.e., 2.00 g of LiAlO<sub>2</sub>, 1 min of mixing (500 rpm) with 25 mL of distilled water, 60 µL (1 × 10<sup>−5</sup> M) of the corresponding collector, 1 min of mixing, 30 µL of frother, and another 1 min of mixing. The flotation was done for 3 min at an air flow rate of 32 mL/min using 250 mL distilled water in daylight at a pH of 10.9 ± 0.3.</p> Full article ">Figure 4
<p>Comparison of the recovery rates of lithium aluminate at different pH values in the presence of the collectors <b>1</b>–<b>3</b> under identical conditions, i.e., 2.00 g of LiAlO<sub>2</sub>, 1 min of mixing (500 rpm) with 25 mL of distilled water, 60 µL (1 × 10<sup>−5</sup> M) of the corresponding collector, 1 min of mixing, 30 µL of frother, and another 1 min of mixing. The flotation was done for 3 min at an air flow rate of 32 mL/min using 250 mL distilled water in daylight.</p> Full article ">Figure 5
<p>Single mineral flotation of lithium aluminate and gehlenite using punicine <b>3</b> as collector at pH 10.9 ± 0.3 for lithium aluminate and pH 8.3 ± 0.3 for gehlenite at different light scenarios. The conditioning was as follows: 120 µL collector, 1 min mixing, 30 µL frother, 1 min mixing, stirring speed 500 rpm, air flow rate of 32 cm<sup>3</sup>/min using 250 mL distilled water, and flotation time of 3 min.</p> Full article ">Figure 6
<p>Single mineral flotation of plasma-pretreated lithium aluminate and the gehlenite-rich material without collector at pH 10.9 ± 0.3 for lithium aluminate and pH 8.3 ± 0.3 for gehlenite as a function of plasma treatment time. Conditioning was as follows: 30 µL frother, 1 min mixing, stirring speed 500 rpm, air flow rate of 32 cm<sup>3</sup>/min using 250 mL distilled water, and flotation time of 3 min.</p> Full article ">Figure 7
<p>Single mineral flotation of the oxidative plasma pretreated lithium aluminate and gehlenite using punicine <b>3</b> as a collector at pH 10.9 ± 0.3 for lithium aluminate, and pH 8.3 for gehlenite as a function of plasma treatment time in min. The conditioning was as follows: 60 µL collector, 1 min mixing 30 µL frother, 1 min mixing, stirring speed of 500 rpm, air flow rate of 32 cm<sup>3</sup>/min using 250 mL distilled water, and applying a flotation time of 3 min.</p> Full article ">Figure 8
<p>Single mineral flotation of the reductive plasma pretreated lithium aluminate in blank and using punicine <b>3</b> as a collector at pH 10.9 ± 0.3 as a function of plasma treatment time in min. The conditioning was as follows: 60 µL collector, 1 min mixing 30 µL frother, 1 min mixing, stirring speed of 500 rpm, air flow rate of 32 cm<sup>3</sup>/min using 250 mL distilled water, and flotation time of 3 min.</p> Full article ">Figure 9
<p>Contact angle on LiAlO<sub>2</sub> before and after flotation with punicine <b>3</b> under identical conditions, i.e., 2.00 g of the mineral, 1 min mixing (500 rpm) with 25 mL distilled water, 120 µL (2 × 10<sup>−5</sup> M) of the corresponding collector, 1 min mixing, 30 µL foaming agent, and 1 min mixing again. Flotation was carried out for 3 min at an air flow rate of 32 mL/min with 250 mL of distilled water in daylight and a pH of 10.9 ± 0.3.</p> Full article ">Figure 10
<p>Zeta potential of pure lithium aluminate, lithium aluminate with 7.2 µL of 42.5 mmol/L punicine <b>1</b>, lithium aluminate with 7.2 µL of 42.5 mmol/L punicine <b>2,</b> and lithium aluminate with 7.2 µL of 42.5 mmol/L punicine <b>3</b> in distilled water and under different pH values.</p> Full article ">Figure 11
<p>FTIR spectra of LiAlO<sub>2</sub> in the presence of 60 µL 42.5 µmol/L punicine <b>3</b> with and without pretreatment with plasma.</p> Full article ">Figure 12
<p>Zeta potential of pure and oxidative plasma-pretreated lithium aluminate, with and without 10 µL of 42.5 mmol/L punicine <b>3</b> at pH 10.9 ± 0.3.</p> Full article ">Scheme 1
<p>Schematic representation of the switchability of punicine with respect to charges and radical status.</p> Full article ">Scheme 2
<p>Intermolecular interactions and complexes of punicine.</p> Full article ">Scheme 3
<p>Synthesis of the punicine derivatives.</p> Full article ">Scheme 4
<p>Reactions of lithium aluminate surfaces with water.</p> Full article ">Scheme 5
<p>Interactions of punicines with lithium aluminum surfaces.</p> Full article ">
Open AccessArticle
Modified Biochar Adsorption Combined with Alkaline Solution Absorption for Sulfur-Containing Odor Gases Removal from Domestic Waste Transfer Stations
by
Wei Wei, Ningjie Wang and Xiaolei Zhang
Separations 2024, 11(12), 361; https://doi.org/10.3390/separations11120361 - 23 Dec 2024
Abstract
Odor emission has become a major issue in waste transfer stations. Hydrogen sulfide, methyl mercaptan (MM), and dimethyl disulfide (DMDS) are the main odorous gases. They have a low odor threshold and are difficult to remove. In this study, pine bark biochar was
[...] Read more.
Odor emission has become a major issue in waste transfer stations. Hydrogen sulfide, methyl mercaptan (MM), and dimethyl disulfide (DMDS) are the main odorous gases. They have a low odor threshold and are difficult to remove. In this study, pine bark biochar was produced and modified with metal ions, including Ni2+, Ti2+, Mn2+, Zn2+, Mg2+, and Cu2+. It was then used for the removal of hydrogen sulfide, methyl mercaptan, and dimethyl disulfide. Among all modifications, the Cu2+ modified biochar showed the best sorption capacity, and the maximum sorption amounts were 20.50 mg/g for H2S, 36.50 mg/g for MM, and 57.98 mg/g for DMDS. To understand the adsorption, BET, SEM, and XPS of the original and modified biochar were performed. This illustrated that modification with Cu2+ increased the surface area and porosity, thus enhancing the adsorption capacity. In the alkaline absorption study, it was found that the removal of the three odor gases increased with the pH increase. Based on the results, a combined process called absorption–adsorption was established to treat the odor gas generated in a local waste transfer station. Thirty-one gas components were detected in the odor gas of the waste transfer station. The process proceeded for 30 days, and these gas components were not found in the effluent during treatment. Regarding H2S, MM, and DMDS, they were not detected even after 90 days. This indicates the high adsorption capacity of the modified biochar toward the three odor gases. In addition, the process is simple and easy to operate. This suggests that it is suitable for treating odor in places where there is no technician, and the odor needs efficient treatment. The study provides a feasible alternative for domestic waste transfer stations to control the odor problem.
Full article
(This article belongs to the Topic Advances in Separation Engineering)
►▼
Show Figures
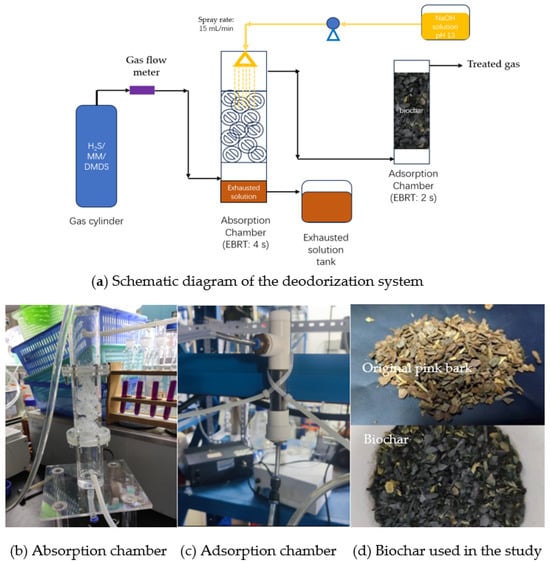
Figure 1
Figure 1
<p>The deodorization system employed for the removal of H<sub>2</sub>S, MM, and DMDS.</p> Full article ">Figure 2
<p>Removal of odor gases by chemical absorption under different conditions: (<b>a</b>) Scrubbing solution pH; (<b>b</b>) Empty bed residence time of the reactor; and (<b>c</b>) NaOH solution dosage.</p> Full article ">Figure 2 Cont.
<p>Removal of odor gases by chemical absorption under different conditions: (<b>a</b>) Scrubbing solution pH; (<b>b</b>) Empty bed residence time of the reactor; and (<b>c</b>) NaOH solution dosage.</p> Full article ">Figure 3
<p>Biochar adsorption capacity: (<b>a</b>) Biochar modified with different metal ions; (<b>b</b>) Biochar modified with various concentrations of Cu<sup>2+</sup>; (<b>c</b>) Cu<sup>2+</sup>-modified biochar obtained at different combustion temperatures.</p> Full article ">Figure 3 Cont.
<p>Biochar adsorption capacity: (<b>a</b>) Biochar modified with different metal ions; (<b>b</b>) Biochar modified with various concentrations of Cu<sup>2+</sup>; (<b>c</b>) Cu<sup>2+</sup>-modified biochar obtained at different combustion temperatures.</p> Full article ">Figure 4
<p>Characteristics of the original and modified biochar: (<b>a</b>) Nitrogen adsorption–desorption curve of biochar; (<b>b</b>) Pore size distribution from 0 to 300 nm; (<b>c</b>) Pore size distribution on a smaller scale compared to (<b>b</b>), which is from 0 to 4 nm.</p> Full article ">Figure 5
<p>SEM analysis of the original and Cu<sup>2+</sup>-modified biochar.</p> Full article ">Figure 5 Cont.
<p>SEM analysis of the original and Cu<sup>2+</sup>-modified biochar.</p> Full article ">Figure 6
<p>The XPS spectra of elements in the modified biochar before and after utilization in adsorption. (<b>a</b>,<b>b</b>) Original biochar with the appearance of observed elements; (<b>c</b>–<b>e</b>) Biochar after utilization in adsorption of H<sub>2</sub>S, MM, and DMDS, respectively.</p> Full article ">
<p>The deodorization system employed for the removal of H<sub>2</sub>S, MM, and DMDS.</p> Full article ">Figure 2
<p>Removal of odor gases by chemical absorption under different conditions: (<b>a</b>) Scrubbing solution pH; (<b>b</b>) Empty bed residence time of the reactor; and (<b>c</b>) NaOH solution dosage.</p> Full article ">Figure 2 Cont.
<p>Removal of odor gases by chemical absorption under different conditions: (<b>a</b>) Scrubbing solution pH; (<b>b</b>) Empty bed residence time of the reactor; and (<b>c</b>) NaOH solution dosage.</p> Full article ">Figure 3
<p>Biochar adsorption capacity: (<b>a</b>) Biochar modified with different metal ions; (<b>b</b>) Biochar modified with various concentrations of Cu<sup>2+</sup>; (<b>c</b>) Cu<sup>2+</sup>-modified biochar obtained at different combustion temperatures.</p> Full article ">Figure 3 Cont.
<p>Biochar adsorption capacity: (<b>a</b>) Biochar modified with different metal ions; (<b>b</b>) Biochar modified with various concentrations of Cu<sup>2+</sup>; (<b>c</b>) Cu<sup>2+</sup>-modified biochar obtained at different combustion temperatures.</p> Full article ">Figure 4
<p>Characteristics of the original and modified biochar: (<b>a</b>) Nitrogen adsorption–desorption curve of biochar; (<b>b</b>) Pore size distribution from 0 to 300 nm; (<b>c</b>) Pore size distribution on a smaller scale compared to (<b>b</b>), which is from 0 to 4 nm.</p> Full article ">Figure 5
<p>SEM analysis of the original and Cu<sup>2+</sup>-modified biochar.</p> Full article ">Figure 5 Cont.
<p>SEM analysis of the original and Cu<sup>2+</sup>-modified biochar.</p> Full article ">Figure 6
<p>The XPS spectra of elements in the modified biochar before and after utilization in adsorption. (<b>a</b>,<b>b</b>) Original biochar with the appearance of observed elements; (<b>c</b>–<b>e</b>) Biochar after utilization in adsorption of H<sub>2</sub>S, MM, and DMDS, respectively.</p> Full article ">
Open AccessArticle
Ion Activity Coefficient of Sodium Halides in Anion-Exchange Polymers: Empirical Model Based on Manning’s Counterion Condensation Theory
by
Guiming Liu and Dandan Gao
Separations 2024, 11(12), 360; https://doi.org/10.3390/separations11120360 - 23 Dec 2024
Abstract
The theory of electrolyte solution provides a precise description of the thermodynamic state and non-ideality of electrolyte solutions, allowing for the accurate prediction of the crystallization separation process of Salt Lake brine. Analogously, we attempt to describe the non-ideality of ions in ion-exchange
[...] Read more.
The theory of electrolyte solution provides a precise description of the thermodynamic state and non-ideality of electrolyte solutions, allowing for the accurate prediction of the crystallization separation process of Salt Lake brine. Analogously, we attempt to describe the non-ideality of ions in ion-exchange polymers based on Manning’s Counterion Condensation Theory, which was originally used to describe the thermodynamics of polyelectrolyte solutions, has amply proven the potential to extend to ion-exchange polymers. In this article, equilibrium solvent and solute concentrations in aminated cross-linked polystyrene AEM were determined experimentally as a function of external NaCl concentration, and ion activity coefficients in the membranes were obtained via a thermodynamic treatment. With the recombination and empirical parameters added to Manning’s model, the ion activity coefficient of NaCl and NaBr in the aminated cross-linked polystyrene AEM can be accurately described in concentration ranges of 0.01 mol·kg−1~3 mol·kg−1. Compared with the original model, the Coefficient of Determination between the improved model and the experimental data was increased from 0.65 to 0.95. The Residual Sum of Squares is reduced by about one order of magnitude, significantly improving the Manning model’s adaptability when applied to AEM.
Full article
(This article belongs to the Special Issue Green and Efficient Separation and Extraction of Salt Lake Resources)
►▼
Show Figures
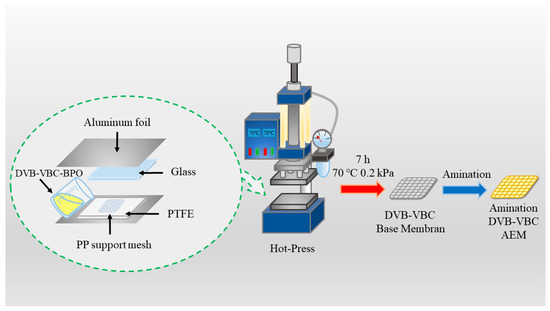
Figure 1
Figure 1
<p>The process for preparing aminated cross-linked polystyrene (ACLP) involves bulk polymerization followed by amination [<a href="#B43-separations-11-00360" class="html-bibr">43</a>].</p> Full article ">Figure 2
<p>The “equilibrium–desorption–exchange” step was employed to measure concentrations within the AEM [<a href="#B10-separations-11-00360" class="html-bibr">10</a>], as illustrated by the different colors in this figure. After the AEM reached swelling equilibrium in the solution, the internal co-ions (Na<sup>+</sup>) and some counter-ions (Cl<sup>−</sup>, Br<sup>−</sup>) contributed by mobile electrolytes could be determined through multiple desorptions of secondary water. Subsequently, the electrostatically bound counter-ions on the fixed groups were exchanged with a 0.1 mol·L<sup>−</sup><sup>1</sup> Na<sub>2</sub>SO<sub>4</sub> solution.</p> Full article ">Figure 3
<p>The influence of external solution molality and cross-linking on the water uptake of ACLP AEMs (excluding the internal PP fiber) is significant. Water uptake decreases with increasing external solution molality and cross-linking. The water uptake values are reported as the mass of water per unit mass of the ion-exchange polymer in the membrane.</p> Full article ">Figure 4
<p>The molal concentrations of co-ions, <math display="inline"><semantics> <mrow> <msubsup> <mrow> <mi mathvariant="italic">m</mi> </mrow> <mrow> <msup> <mrow> <mi>Na</mi> </mrow> <mrow> <mo>+</mo> </mrow> </msup> </mrow> <mrow> <mi mathvariant="normal">m</mi> </mrow> </msubsup> </mrow> </semantics></math>, and counter-ions, <math display="inline"><semantics> <mrow> <msubsup> <mrow> <mi mathvariant="italic">m</mi> </mrow> <mrow> <msup> <mrow> <mi mathvariant="normal">X</mi> </mrow> <mrow> <mo>−</mo> </mrow> </msup> </mrow> <mrow> <mi mathvariant="normal">m</mi> </mrow> </msubsup> <mtext> </mtext> </mrow> </semantics></math> (X = Cl, Br), in the anion-exchange polymer are presented as a function of external electrolyte concentration for NaCl (<b>a</b>,<b>b</b>) and NaBr (<b>c</b>,<b>d</b>).</p> Full article ">Figure 5
<p>The influence of external electrolyte concentration <math display="inline"><semantics> <mrow> <msubsup> <mrow> <mi mathvariant="italic">m</mi> </mrow> <mrow> <mi>NaX</mi> </mrow> <mrow> <mi mathvariant="normal">s</mi> </mrow> </msubsup> </mrow> </semantics></math> on the activity coefficients of the membrane in NaCl (<b>a</b>) and NaBr (<b>b</b>) is presented and obtained experimentally via Equation (10). Additionally, the respective ionic activity coefficients of the co-ions <math display="inline"><semantics> <mrow> <msubsup> <mrow> <mo mathvariant="italic">γ</mo> </mrow> <mrow> <msup> <mrow> <mi>Na</mi> </mrow> <mrow> <mo>+</mo> </mrow> </msup> </mrow> <mrow> <mi mathvariant="normal">m</mi> </mrow> </msubsup> </mrow> </semantics></math> (<b>c</b>) and counter-ions <math display="inline"><semantics> <mrow> <msubsup> <mrow> <mo mathvariant="italic">γ</mo> </mrow> <mrow> <msup> <mrow> <mi mathvariant="normal">X</mi> </mrow> <mrow> <mo>-</mo> </mrow> </msup> </mrow> <mrow> <mi mathvariant="normal">m</mi> </mrow> </msubsup> </mrow> </semantics></math> (<b>d</b>) in the membrane were determined experimentally using Equation (7).</p> Full article ">Figure 6
<p>(<b>a</b>) illustrates the scenarios described by Manning’s theory, specifically for the cases where <span class="html-italic">b</span> > <span class="html-italic">λ</span><sub>b</sub> and <span class="html-italic">b</span> < <span class="html-italic">λ</span><sub>b</sub>. In the case where <span class="html-italic">b</span> < <span class="html-italic">λ</span><sub>b</sub>, the overlap of the Bjerrum length creates a localized energy minimum region where counterions are likely to reside, leading to “counterion condensation” [<a href="#B28-separations-11-00360" class="html-bibr">28</a>]. (<b>b</b>) mathematically presents the left and right limits predicted by the Manning model under this assumption, as expressed by Equation (13).</p> Full article ">Figure 7
<p>(<b>a</b>) In the assumptions of Manning’s theory, the polymer in a dilute polyelectrolyte solution is modeled as a chain of linear charges, with the solvent and counter-ions shielded from the effects of other nearby charge chains [<a href="#B28-separations-11-00360" class="html-bibr">28</a>]. (<b>b</b>) The swollen ion-exchange membrane cannot be regarded as a dilute solution, and obviously cannot be approximated as a linear chain charge. The Rayleig–Flory model of an irregular free link chain is closer to the real situation [<a href="#B47-separations-11-00360" class="html-bibr">47</a>], The resulting line charge density deviation is corrected by <span class="html-italic">α</span>. (<b>c</b>) The distance between the chain charges of the swelling ion-exchange membrane is much smaller than that of the dilute polyelectrolyte solution, which makes it difficult for the solvent and counter-ions to shield the nearby chain. This shielding may gradually fail at low concentrations, which is reflected by the electric field superposition of multi-segment chains, and leads to the further decrease in the ion activity coefficient that the deviation is corrected by <span class="html-italic">β</span>.</p> Full article ">Figure 8
<p>Ion activity coefficients in ion-exchange membranes as a function of external NaCl concentration obtained experimentally (ACLP-2% (●), ACLP-3% (◆), and ACLP-4% (■)) and predicted by Manning’s limiting laws (ACLP-2% (red dashed line), ACLP-3% (green dashed line), and ACLP-4% (black dash-dotted line)). As shown in (<b>a</b>), (<b>b</b>), (<b>c</b>), respectively, represent the introduction of correction parameter <span class="html-italic">α</span>; and the prediction results after introducing correction parameters <span class="html-italic">α</span> and <span class="html-italic">β</span>.</p> Full article ">Figure 9
<p>Ion activity coefficients in ion-exchange membranes as a function of external NaBr concentration obtained experimentally (ACLP-2% (●), ACLP-3% (◆), and ACLP-4% (■)) and predicted by Manning’s limiting laws (ACLP-2% (yellow dashed line), ACLP-3% (teal dashed line), and ACLP-4% (indigo dash-dotted line)). As shown in (<b>a</b>), (<b>b</b>), (<b>c</b>), respectively, represent the introduction of correction parameter <span class="html-italic">α</span>; And the prediction results after introducing correction parameters <span class="html-italic">α</span> and <span class="html-italic">β</span>.</p> Full article ">Figure 10
<p>The empirical expression of <span class="html-italic">β</span> as a function of <math display="inline"><semantics> <mrow> <mrow> <mi>ln</mi> <mo>(</mo> </mrow> <msubsup> <mrow> <msubsup> <mrow> <mi mathvariant="italic">m</mi> </mrow> <mrow> <mi mathvariant="normal">A</mi> </mrow> <mrow> <mi mathvariant="normal">m</mi> </mrow> </msubsup> <mo>/</mo> <mi mathvariant="italic">m</mi> </mrow> <mrow> <mi mathvariant="normal">s</mi> </mrow> <mrow> <mi mathvariant="normal">m</mi> </mrow> </msubsup> <mo>)</mo> </mrow> </semantics></math> is obtained by fitting the experimental data of ACLP-2%, ACLP-3%, and ACLP-4% equilibrium with NaCl solution. According to the hypothesis of the modified model, the mobile salt concentration affects the shielding of the surrounding chain charge. When the value of <math display="inline"><semantics> <mrow> <msubsup> <mrow> <mi mathvariant="italic">m</mi> </mrow> <mrow> <mi mathvariant="normal">s</mi> </mrow> <mrow> <mi mathvariant="normal">m</mi> </mrow> </msubsup> </mrow> </semantics></math> decreases, the value of <math display="inline"><semantics> <mrow> <msubsup> <mrow> <msubsup> <mrow> <mi mathvariant="italic">m</mi> </mrow> <mrow> <mi mathvariant="normal">A</mi> </mrow> <mrow> <mi mathvariant="normal">m</mi> </mrow> </msubsup> <mo>/</mo> <mi mathvariant="italic">m</mi> </mrow> <mrow> <mi mathvariant="normal">s</mi> </mrow> <mrow> <mi mathvariant="normal">m</mi> </mrow> </msubsup> </mrow> </semantics></math> increases. In this case, the shielding gradually weakens and shows the chain electric field superposition scenario shown in <a href="#separations-11-00360-f007" class="html-fig">Figure 7</a>c, which further reduces the ion activity coefficient, the resulting reduction factor is captured by the correction factor <span class="html-italic">β</span>; However, in high concentration solutions, the value of <math display="inline"><semantics> <mrow> <msubsup> <mrow> <mi mathvariant="italic">m</mi> </mrow> <mrow> <mi mathvariant="normal">s</mi> </mrow> <mrow> <mi mathvariant="normal">m</mi> </mrow> </msubsup> </mrow> </semantics></math> will be very large, providing enough shielding for nearby chain charges and making the value of <math display="inline"><semantics> <mrow> <msubsup> <mrow> <msubsup> <mrow> <mi mathvariant="italic">m</mi> </mrow> <mrow> <mi mathvariant="normal">A</mi> </mrow> <mrow> <mi mathvariant="normal">m</mi> </mrow> </msubsup> <mo>/</mo> <mi mathvariant="italic">m</mi> </mrow> <mrow> <mi mathvariant="normal">s</mi> </mrow> <mrow> <mi mathvariant="normal">m</mi> </mrow> </msubsup> </mrow> </semantics></math> very low, in which case <span class="html-italic">β</span> = 1 and returning to the situation of <a href="#separations-11-00360-f007" class="html-fig">Figure 7</a>b.</p> Full article ">Figure 11
<p>Ion activity coefficients for NaCl in anion- and cation-exchange resins [AR103 (<b>a</b>) and CR61 (<b>b</b>)]; Circular points denote values obtained experimentally, dashed lines denote values obtained by Manning’s original model (The yellow and blue regions represent the acceptable prediction range of the original model); and the solid line represents the predicted value of the improved model as Equation (26) after introducing the empirical parameters, the expression of parameter β can obtained by nonlinear curve fitting of experimental data, using similar form of Equation (25).</p> Full article ">
<p>The process for preparing aminated cross-linked polystyrene (ACLP) involves bulk polymerization followed by amination [<a href="#B43-separations-11-00360" class="html-bibr">43</a>].</p> Full article ">Figure 2
<p>The “equilibrium–desorption–exchange” step was employed to measure concentrations within the AEM [<a href="#B10-separations-11-00360" class="html-bibr">10</a>], as illustrated by the different colors in this figure. After the AEM reached swelling equilibrium in the solution, the internal co-ions (Na<sup>+</sup>) and some counter-ions (Cl<sup>−</sup>, Br<sup>−</sup>) contributed by mobile electrolytes could be determined through multiple desorptions of secondary water. Subsequently, the electrostatically bound counter-ions on the fixed groups were exchanged with a 0.1 mol·L<sup>−</sup><sup>1</sup> Na<sub>2</sub>SO<sub>4</sub> solution.</p> Full article ">Figure 3
<p>The influence of external solution molality and cross-linking on the water uptake of ACLP AEMs (excluding the internal PP fiber) is significant. Water uptake decreases with increasing external solution molality and cross-linking. The water uptake values are reported as the mass of water per unit mass of the ion-exchange polymer in the membrane.</p> Full article ">Figure 4
<p>The molal concentrations of co-ions, <math display="inline"><semantics> <mrow> <msubsup> <mrow> <mi mathvariant="italic">m</mi> </mrow> <mrow> <msup> <mrow> <mi>Na</mi> </mrow> <mrow> <mo>+</mo> </mrow> </msup> </mrow> <mrow> <mi mathvariant="normal">m</mi> </mrow> </msubsup> </mrow> </semantics></math>, and counter-ions, <math display="inline"><semantics> <mrow> <msubsup> <mrow> <mi mathvariant="italic">m</mi> </mrow> <mrow> <msup> <mrow> <mi mathvariant="normal">X</mi> </mrow> <mrow> <mo>−</mo> </mrow> </msup> </mrow> <mrow> <mi mathvariant="normal">m</mi> </mrow> </msubsup> <mtext> </mtext> </mrow> </semantics></math> (X = Cl, Br), in the anion-exchange polymer are presented as a function of external electrolyte concentration for NaCl (<b>a</b>,<b>b</b>) and NaBr (<b>c</b>,<b>d</b>).</p> Full article ">Figure 5
<p>The influence of external electrolyte concentration <math display="inline"><semantics> <mrow> <msubsup> <mrow> <mi mathvariant="italic">m</mi> </mrow> <mrow> <mi>NaX</mi> </mrow> <mrow> <mi mathvariant="normal">s</mi> </mrow> </msubsup> </mrow> </semantics></math> on the activity coefficients of the membrane in NaCl (<b>a</b>) and NaBr (<b>b</b>) is presented and obtained experimentally via Equation (10). Additionally, the respective ionic activity coefficients of the co-ions <math display="inline"><semantics> <mrow> <msubsup> <mrow> <mo mathvariant="italic">γ</mo> </mrow> <mrow> <msup> <mrow> <mi>Na</mi> </mrow> <mrow> <mo>+</mo> </mrow> </msup> </mrow> <mrow> <mi mathvariant="normal">m</mi> </mrow> </msubsup> </mrow> </semantics></math> (<b>c</b>) and counter-ions <math display="inline"><semantics> <mrow> <msubsup> <mrow> <mo mathvariant="italic">γ</mo> </mrow> <mrow> <msup> <mrow> <mi mathvariant="normal">X</mi> </mrow> <mrow> <mo>-</mo> </mrow> </msup> </mrow> <mrow> <mi mathvariant="normal">m</mi> </mrow> </msubsup> </mrow> </semantics></math> (<b>d</b>) in the membrane were determined experimentally using Equation (7).</p> Full article ">Figure 6
<p>(<b>a</b>) illustrates the scenarios described by Manning’s theory, specifically for the cases where <span class="html-italic">b</span> > <span class="html-italic">λ</span><sub>b</sub> and <span class="html-italic">b</span> < <span class="html-italic">λ</span><sub>b</sub>. In the case where <span class="html-italic">b</span> < <span class="html-italic">λ</span><sub>b</sub>, the overlap of the Bjerrum length creates a localized energy minimum region where counterions are likely to reside, leading to “counterion condensation” [<a href="#B28-separations-11-00360" class="html-bibr">28</a>]. (<b>b</b>) mathematically presents the left and right limits predicted by the Manning model under this assumption, as expressed by Equation (13).</p> Full article ">Figure 7
<p>(<b>a</b>) In the assumptions of Manning’s theory, the polymer in a dilute polyelectrolyte solution is modeled as a chain of linear charges, with the solvent and counter-ions shielded from the effects of other nearby charge chains [<a href="#B28-separations-11-00360" class="html-bibr">28</a>]. (<b>b</b>) The swollen ion-exchange membrane cannot be regarded as a dilute solution, and obviously cannot be approximated as a linear chain charge. The Rayleig–Flory model of an irregular free link chain is closer to the real situation [<a href="#B47-separations-11-00360" class="html-bibr">47</a>], The resulting line charge density deviation is corrected by <span class="html-italic">α</span>. (<b>c</b>) The distance between the chain charges of the swelling ion-exchange membrane is much smaller than that of the dilute polyelectrolyte solution, which makes it difficult for the solvent and counter-ions to shield the nearby chain. This shielding may gradually fail at low concentrations, which is reflected by the electric field superposition of multi-segment chains, and leads to the further decrease in the ion activity coefficient that the deviation is corrected by <span class="html-italic">β</span>.</p> Full article ">Figure 8
<p>Ion activity coefficients in ion-exchange membranes as a function of external NaCl concentration obtained experimentally (ACLP-2% (●), ACLP-3% (◆), and ACLP-4% (■)) and predicted by Manning’s limiting laws (ACLP-2% (red dashed line), ACLP-3% (green dashed line), and ACLP-4% (black dash-dotted line)). As shown in (<b>a</b>), (<b>b</b>), (<b>c</b>), respectively, represent the introduction of correction parameter <span class="html-italic">α</span>; and the prediction results after introducing correction parameters <span class="html-italic">α</span> and <span class="html-italic">β</span>.</p> Full article ">Figure 9
<p>Ion activity coefficients in ion-exchange membranes as a function of external NaBr concentration obtained experimentally (ACLP-2% (●), ACLP-3% (◆), and ACLP-4% (■)) and predicted by Manning’s limiting laws (ACLP-2% (yellow dashed line), ACLP-3% (teal dashed line), and ACLP-4% (indigo dash-dotted line)). As shown in (<b>a</b>), (<b>b</b>), (<b>c</b>), respectively, represent the introduction of correction parameter <span class="html-italic">α</span>; And the prediction results after introducing correction parameters <span class="html-italic">α</span> and <span class="html-italic">β</span>.</p> Full article ">Figure 10
<p>The empirical expression of <span class="html-italic">β</span> as a function of <math display="inline"><semantics> <mrow> <mrow> <mi>ln</mi> <mo>(</mo> </mrow> <msubsup> <mrow> <msubsup> <mrow> <mi mathvariant="italic">m</mi> </mrow> <mrow> <mi mathvariant="normal">A</mi> </mrow> <mrow> <mi mathvariant="normal">m</mi> </mrow> </msubsup> <mo>/</mo> <mi mathvariant="italic">m</mi> </mrow> <mrow> <mi mathvariant="normal">s</mi> </mrow> <mrow> <mi mathvariant="normal">m</mi> </mrow> </msubsup> <mo>)</mo> </mrow> </semantics></math> is obtained by fitting the experimental data of ACLP-2%, ACLP-3%, and ACLP-4% equilibrium with NaCl solution. According to the hypothesis of the modified model, the mobile salt concentration affects the shielding of the surrounding chain charge. When the value of <math display="inline"><semantics> <mrow> <msubsup> <mrow> <mi mathvariant="italic">m</mi> </mrow> <mrow> <mi mathvariant="normal">s</mi> </mrow> <mrow> <mi mathvariant="normal">m</mi> </mrow> </msubsup> </mrow> </semantics></math> decreases, the value of <math display="inline"><semantics> <mrow> <msubsup> <mrow> <msubsup> <mrow> <mi mathvariant="italic">m</mi> </mrow> <mrow> <mi mathvariant="normal">A</mi> </mrow> <mrow> <mi mathvariant="normal">m</mi> </mrow> </msubsup> <mo>/</mo> <mi mathvariant="italic">m</mi> </mrow> <mrow> <mi mathvariant="normal">s</mi> </mrow> <mrow> <mi mathvariant="normal">m</mi> </mrow> </msubsup> </mrow> </semantics></math> increases. In this case, the shielding gradually weakens and shows the chain electric field superposition scenario shown in <a href="#separations-11-00360-f007" class="html-fig">Figure 7</a>c, which further reduces the ion activity coefficient, the resulting reduction factor is captured by the correction factor <span class="html-italic">β</span>; However, in high concentration solutions, the value of <math display="inline"><semantics> <mrow> <msubsup> <mrow> <mi mathvariant="italic">m</mi> </mrow> <mrow> <mi mathvariant="normal">s</mi> </mrow> <mrow> <mi mathvariant="normal">m</mi> </mrow> </msubsup> </mrow> </semantics></math> will be very large, providing enough shielding for nearby chain charges and making the value of <math display="inline"><semantics> <mrow> <msubsup> <mrow> <msubsup> <mrow> <mi mathvariant="italic">m</mi> </mrow> <mrow> <mi mathvariant="normal">A</mi> </mrow> <mrow> <mi mathvariant="normal">m</mi> </mrow> </msubsup> <mo>/</mo> <mi mathvariant="italic">m</mi> </mrow> <mrow> <mi mathvariant="normal">s</mi> </mrow> <mrow> <mi mathvariant="normal">m</mi> </mrow> </msubsup> </mrow> </semantics></math> very low, in which case <span class="html-italic">β</span> = 1 and returning to the situation of <a href="#separations-11-00360-f007" class="html-fig">Figure 7</a>b.</p> Full article ">Figure 11
<p>Ion activity coefficients for NaCl in anion- and cation-exchange resins [AR103 (<b>a</b>) and CR61 (<b>b</b>)]; Circular points denote values obtained experimentally, dashed lines denote values obtained by Manning’s original model (The yellow and blue regions represent the acceptable prediction range of the original model); and the solid line represents the predicted value of the improved model as Equation (26) after introducing the empirical parameters, the expression of parameter β can obtained by nonlinear curve fitting of experimental data, using similar form of Equation (25).</p> Full article ">
Open AccessArticle
Unveiling the Floral Scent Dynamics of Calamondin (Citrus × microcarpa) Across Developmental Stages
by
Yiwei Chen, Zhiqing Liang, Shiyu Chen, Fulong Yan, Jingjuan He, Yiwei Zhou and Ting Gao
Separations 2024, 11(12), 359; https://doi.org/10.3390/separations11120359 - 23 Dec 2024
Abstract
The calamondin (Citrus × microcarpa) is highly valued for its ornamental appeal and rich aromatic compounds, making it suitable for therapeutic gardens and widely applicable in the cosmetics, food, pharmaceutical, and perfume industries. Despite its importance, there is a lack of
[...] Read more.
The calamondin (Citrus × microcarpa) is highly valued for its ornamental appeal and rich aromatic compounds, making it suitable for therapeutic gardens and widely applicable in the cosmetics, food, pharmaceutical, and perfume industries. Despite its importance, there is a lack of research on its floral volatiles. This study utilized headspace solid-phase microextraction gas chromatography–mass spectrometry (HS–SPME–GC–MS) to detect the volatile organic compounds (VOCs) of calamondin at different floral developmental stages: bud (BS), half-open (HS), full bloom (FS), and senescence (SS). Multivariate statistical analysis was employed to elucidate the aromatic characteristics. The results identified 67 VOCs across the four stages, including forty-eight terpenoids, six esters, five aromatics, four aldehydes, one olefin, one alcohol, and two others. Thirty-three VOCs were common across all stages, while BS, HS, FS, and SS had three, three, four, and nine unique VOCs, respectively. The total VOC content increased initially and then decreased as the flowers developed, with terpenoids being the predominant compounds, accounting for over 90% of the total emissions at all stages. Principal component analysis and hierarchical cluster analysis confirmed significant differences in VOC profiles at different stages. Partial least squares discriminant analysis identified five VOCs with variable importance in projection (VIP) values greater than one, including limonene, linalool, β-pinene, germacrene D, and β-ocimene, indicating their varying emission levels across stages. These findings enhance our understanding of the VOC characteristics of calamondin flowers and provide a scientific basis for further ornamental and industrial applications.
Full article
(This article belongs to the Special Issue Research Progress for Isolation of Plant Active Compounds)
►▼
Show Figures
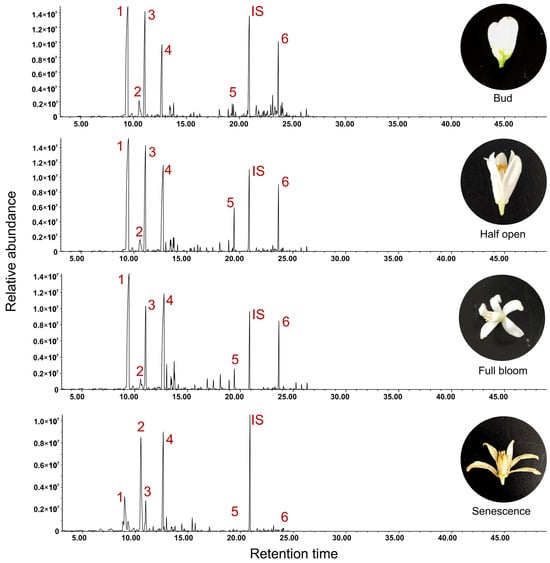
Figure 1
Figure 1
<p>Total Ion Chromatograms (TIC) of calamondin’s floral scent at four different developmental stages detected by HS-SPME-GC-MS. The highlighted numbers indicate the main identified peak areas. 1: <span class="html-italic">β</span>-Pinene; 2: Limonene; 3: <span class="html-italic">β</span>-Ocimene; 4: Linalool; 5: Methyl anthranilate; 6: <span class="html-italic">α</span>-Farnesene. IS: Internal standard, ethyl caproate.</p> Full article ">Figure 2
<p>Identification of the number of different categories of VOCs at four developmental stages. (<b>A</b>) Number of different VOC categories in floral scents at four developmental stages; (<b>B</b>) Composition of 67 VOCs by compound category; (<b>C</b>) Venn diagram analysis of unique and common VOCs in floral scents at different stages.</p> Full article ">Figure 3
<p>Comparative analysis of the relative total VOC content and the percentage of different VOC categories at four different flowering stages. (<b>A</b>) Comparative analysis of total VOC content. Different lowercase letters indicate significant differences at the <span class="html-italic">p</span> < 0.05 level. (<b>B</b>–<b>E</b>) Relative content percentages of different VOC categories at BS, HS, FS, and SS stages, respectively.</p> Full article ">Figure 4
<p>PCA analysis based on the relative content of 67 VOCs.</p> Full article ">Figure 5
<p>HCA heatmap analysis based on the relative content of 67 VOCs.</p> Full article ">Figure 6
<p>K-means analysis based on the relative content of 67 VOCs. The black line elucidates the trend in the relative content of the specific VOCs across four floral development stages. Simultaneously, the colored lines represent the variation in the relative abundance of diverse VOCs within different groups, spanning four floral development stages.</p> Full article ">Figure 7
<p>Top 10 VOCs with the highest VIP values based on PLS-DA analysis. The pink color means the compound’s value of VIP greater than 1, and the blue color means the compound’s value of VIP less than 1.</p> Full article ">
<p>Total Ion Chromatograms (TIC) of calamondin’s floral scent at four different developmental stages detected by HS-SPME-GC-MS. The highlighted numbers indicate the main identified peak areas. 1: <span class="html-italic">β</span>-Pinene; 2: Limonene; 3: <span class="html-italic">β</span>-Ocimene; 4: Linalool; 5: Methyl anthranilate; 6: <span class="html-italic">α</span>-Farnesene. IS: Internal standard, ethyl caproate.</p> Full article ">Figure 2
<p>Identification of the number of different categories of VOCs at four developmental stages. (<b>A</b>) Number of different VOC categories in floral scents at four developmental stages; (<b>B</b>) Composition of 67 VOCs by compound category; (<b>C</b>) Venn diagram analysis of unique and common VOCs in floral scents at different stages.</p> Full article ">Figure 3
<p>Comparative analysis of the relative total VOC content and the percentage of different VOC categories at four different flowering stages. (<b>A</b>) Comparative analysis of total VOC content. Different lowercase letters indicate significant differences at the <span class="html-italic">p</span> < 0.05 level. (<b>B</b>–<b>E</b>) Relative content percentages of different VOC categories at BS, HS, FS, and SS stages, respectively.</p> Full article ">Figure 4
<p>PCA analysis based on the relative content of 67 VOCs.</p> Full article ">Figure 5
<p>HCA heatmap analysis based on the relative content of 67 VOCs.</p> Full article ">Figure 6
<p>K-means analysis based on the relative content of 67 VOCs. The black line elucidates the trend in the relative content of the specific VOCs across four floral development stages. Simultaneously, the colored lines represent the variation in the relative abundance of diverse VOCs within different groups, spanning four floral development stages.</p> Full article ">Figure 7
<p>Top 10 VOCs with the highest VIP values based on PLS-DA analysis. The pink color means the compound’s value of VIP greater than 1, and the blue color means the compound’s value of VIP less than 1.</p> Full article ">
Open AccessArticle
Metal Sulfide Nanoparticles as Sphalerite Surface Activators to Improve Zinc Recovery Through Flotation Process
by
Delia Monserrat Ávila-Márquez, Alien Blanco-Flores, Helen Paola Toledo-Jaldin, Maribel González Torres, Alfredo Rafael Vilchis-Nestor, Iván Alejandro Reyes Domínguez and Ramiro de Aquino García
Separations 2024, 11(12), 358; https://doi.org/10.3390/separations11120358 - 22 Dec 2024
Abstract
CuS nanoparticles (Np) were synthesized and deposited on synthetic sphalerite (SP) using two different methods. Two nanoparticle products were obtained on the surface of SP, Np1 and Np2, resulting in two active materials (Np1-S
[...] Read more.
CuS nanoparticles (Np) were synthesized and deposited on synthetic sphalerite (SP) using two different methods. Two nanoparticle products were obtained on the surface of SP, Np1 and Np2, resulting in two active materials (Np1-SP and Np2-SP) with specific characteristics. Nanoparticles and active materials were characterized by TEM, XRD, SEM, and XPS. The collectors PAX and SIPX were adsorbed on Np1-SP and Np2-SP to determine the adsorption capacity. Method 1 provides a higher quantity of nanoparticles on SP, which allows for the adsorption of a higher amount of SIPX. Method 1 was used to deposit nanoparticles on two natural sphalerites (SN) with different iron contents. SN, unlike SP, can be used to test nanoparticle activation results in microflotation experiments. SN was activated with nanoparticles (Np1-SN) and using the traditional method (Cu-SN). The recovery of 75% of zinc using the microflotation process suggests that the hydrophobicity of Np1-SN is higher than that of Cu-SN. Nanoparticles improve the hydrophobicity of SN compared to the traditional activation used in the mining industry. These results suggest that using nanoparticles is an excellent option to activate minerals in flotation processes, decreasing the consumption of reagents and helping to mitigate negative impacts on the environment.
Full article
(This article belongs to the Section Materials in Separation Science)
►▼
Show Figures
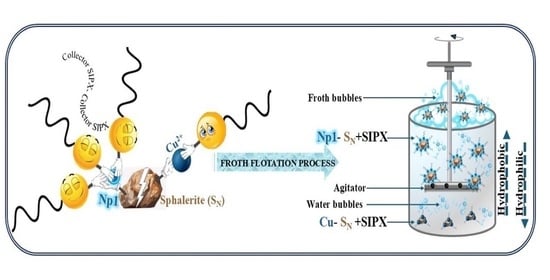
Graphical abstract
Graphical abstract
Full article ">Figure 1
<p>Activation process with the deposition of CuS nanoparticles synthesized by (<b>a</b>) M1 and (<b>b</b>) by M2.</p> Full article ">Figure 2
<p>XRD patterns of <b>S<sub>P</sub></b> and <b>Np1-S<sub>P</sub></b> and <b>Np2-S<sub>P</sub></b>.</p> Full article ">Figure 3
<p>SEM micrographs of <b>Np1-S<sub>P</sub></b> (<b>c</b>,<b>d</b>) and <b>Np2-S<sub>P</sub></b> (<b>a</b>,<b>b</b>,<b>e</b>,<b>f</b>).</p> Full article ">Figure 4
<p>EDS spectra of <b>Np1-S<sub>P</sub></b> using Na<sub>2</sub>S (<b>a</b>) and <b>Np2-S<sub>P</sub></b> using thiourea (<b>b</b>).</p> Full article ">Figure 5
<p>TEM micrographs of <b>Np1-S<sub>P</sub></b> (<b>a</b>) and particle size histogram (<b>b</b>) and <b>Np2-S<sub>P</sub></b> (<b>c</b>) and particle size histogram (<b>d</b>).</p> Full article ">Figure 6
<p>Adsorption kinetics of (<b>a</b>) SIPX and (<b>b</b>) PAX collectors onto <b>Np1-S<sub>P</sub></b>, <b>Np2-S<sub>P</sub></b>, <b>Cu-S<sub>P</sub></b> and (<b>c</b>) adsorption of the SIPX collector onto <b>Np1</b>, <b>Np2</b>, <b>Np1-S<sub>P</sub></b>, <b>Np2-S<sub>P</sub></b>, <b>Cu-S<sub>P</sub></b>.</p> Full article ">Figure 7
<p>XPS survey spectra of <b>Np1-S<sub>P</sub></b> (<b>a</b>), spectra of Zn 2p (<b>b</b>), Zn2p comparation for <b>S<sub>P</sub></b> and <b>Np1-S<sub>P</sub></b> (<b>c</b>), S 2p (<b>d</b>), S2p comparation for <b>S<sub>P</sub></b> and <b>Np1-S<sub>P</sub></b> (<b>e</b>), Cu 2p (<b>f</b>) and O1s (<b>g</b>).</p> Full article ">Figure 7 Cont.
<p>XPS survey spectra of <b>Np1-S<sub>P</sub></b> (<b>a</b>), spectra of Zn 2p (<b>b</b>), Zn2p comparation for <b>S<sub>P</sub></b> and <b>Np1-S<sub>P</sub></b> (<b>c</b>), S 2p (<b>d</b>), S2p comparation for <b>S<sub>P</sub></b> and <b>Np1-S<sub>P</sub></b> (<b>e</b>), Cu 2p (<b>f</b>) and O1s (<b>g</b>).</p> Full article ">Figure 7 Cont.
<p>XPS survey spectra of <b>Np1-S<sub>P</sub></b> (<b>a</b>), spectra of Zn 2p (<b>b</b>), Zn2p comparation for <b>S<sub>P</sub></b> and <b>Np1-S<sub>P</sub></b> (<b>c</b>), S 2p (<b>d</b>), S2p comparation for <b>S<sub>P</sub></b> and <b>Np1-S<sub>P</sub></b> (<b>e</b>), Cu 2p (<b>f</b>) and O1s (<b>g</b>).</p> Full article ">Figure 8
<p>Mechanism to deposition of <b>Np1</b> and collector adsorption onto <b>Np1-S<sub>P</sub></b>.</p> Full article ">Figure 9
<p>Microflotation results of traditionally activated materials and CuS nanoparticles.</p> Full article ">
Full article ">Figure 1
<p>Activation process with the deposition of CuS nanoparticles synthesized by (<b>a</b>) M1 and (<b>b</b>) by M2.</p> Full article ">Figure 2
<p>XRD patterns of <b>S<sub>P</sub></b> and <b>Np1-S<sub>P</sub></b> and <b>Np2-S<sub>P</sub></b>.</p> Full article ">Figure 3
<p>SEM micrographs of <b>Np1-S<sub>P</sub></b> (<b>c</b>,<b>d</b>) and <b>Np2-S<sub>P</sub></b> (<b>a</b>,<b>b</b>,<b>e</b>,<b>f</b>).</p> Full article ">Figure 4
<p>EDS spectra of <b>Np1-S<sub>P</sub></b> using Na<sub>2</sub>S (<b>a</b>) and <b>Np2-S<sub>P</sub></b> using thiourea (<b>b</b>).</p> Full article ">Figure 5
<p>TEM micrographs of <b>Np1-S<sub>P</sub></b> (<b>a</b>) and particle size histogram (<b>b</b>) and <b>Np2-S<sub>P</sub></b> (<b>c</b>) and particle size histogram (<b>d</b>).</p> Full article ">Figure 6
<p>Adsorption kinetics of (<b>a</b>) SIPX and (<b>b</b>) PAX collectors onto <b>Np1-S<sub>P</sub></b>, <b>Np2-S<sub>P</sub></b>, <b>Cu-S<sub>P</sub></b> and (<b>c</b>) adsorption of the SIPX collector onto <b>Np1</b>, <b>Np2</b>, <b>Np1-S<sub>P</sub></b>, <b>Np2-S<sub>P</sub></b>, <b>Cu-S<sub>P</sub></b>.</p> Full article ">Figure 7
<p>XPS survey spectra of <b>Np1-S<sub>P</sub></b> (<b>a</b>), spectra of Zn 2p (<b>b</b>), Zn2p comparation for <b>S<sub>P</sub></b> and <b>Np1-S<sub>P</sub></b> (<b>c</b>), S 2p (<b>d</b>), S2p comparation for <b>S<sub>P</sub></b> and <b>Np1-S<sub>P</sub></b> (<b>e</b>), Cu 2p (<b>f</b>) and O1s (<b>g</b>).</p> Full article ">Figure 7 Cont.
<p>XPS survey spectra of <b>Np1-S<sub>P</sub></b> (<b>a</b>), spectra of Zn 2p (<b>b</b>), Zn2p comparation for <b>S<sub>P</sub></b> and <b>Np1-S<sub>P</sub></b> (<b>c</b>), S 2p (<b>d</b>), S2p comparation for <b>S<sub>P</sub></b> and <b>Np1-S<sub>P</sub></b> (<b>e</b>), Cu 2p (<b>f</b>) and O1s (<b>g</b>).</p> Full article ">Figure 7 Cont.
<p>XPS survey spectra of <b>Np1-S<sub>P</sub></b> (<b>a</b>), spectra of Zn 2p (<b>b</b>), Zn2p comparation for <b>S<sub>P</sub></b> and <b>Np1-S<sub>P</sub></b> (<b>c</b>), S 2p (<b>d</b>), S2p comparation for <b>S<sub>P</sub></b> and <b>Np1-S<sub>P</sub></b> (<b>e</b>), Cu 2p (<b>f</b>) and O1s (<b>g</b>).</p> Full article ">Figure 8
<p>Mechanism to deposition of <b>Np1</b> and collector adsorption onto <b>Np1-S<sub>P</sub></b>.</p> Full article ">Figure 9
<p>Microflotation results of traditionally activated materials and CuS nanoparticles.</p> Full article ">
Open AccessArticle
Innovative Nafion- and Lignin-Based Cation Exchange Materials Against Standard Resins for the Removal of Heavy Metals During Water Treatment
by
Sara Bergamasco, Luis Alexander Hein, Laura Silvestri, Robert Hartmann, Giampiero Menegatti, Alfonso Pozio and Antonio Rinaldi
Separations 2024, 11(12), 357; https://doi.org/10.3390/separations11120357 - 21 Dec 2024
Abstract
The contamination of water by heavy metals poses an escalating risk to human health and the environment, underscoring the critical need for efficient removal methods to secure safe water resources. This study evaluated the performance of four cationic exchange materials (labeled “PS—DVB”, “PA—DVB”,
[...] Read more.
The contamination of water by heavy metals poses an escalating risk to human health and the environment, underscoring the critical need for efficient removal methods to secure safe water resources. This study evaluated the performance of four cationic exchange materials (labeled “PS—DVB”, “PA—DVB”, “TFSA”, and “OGL”) in removing or harvesting metals such as copper, silver, lead, cobalt, and nickel from aqueous solutions, several of which are precious and/or classified as Critical Raw Materials (CRMs) due to their economic importance and supply risk. The objective was to screen and benchmark the four ion exchange materials for water treatment applications by investigating their metal sequestration capacities. Experiments were conducted using synthetic solutions with controlled metal concentrations, analyzed through ICP-OES, and supported by kinetic modeling. The adsorption capacities (qe) obtained experimentally were compared with those predicted by pseudo-first-order and pseudo-second-order models. This methodology enables high precision and reproducibility, validating its applicability for assessing ion exchange performance. The results indicated that PS—DVB and PA—DVB resins proved to be of “wide range”, exhibiting high efficacy for most of the metals tested, including CRM-designated ones, and suggesting their suitability for water purification. Additionally, the second-life Nafion-based “TFSA” material demonstrated commendable performance, highlighting its potential as a viable and technologically advanced alternative in water treatment. Lastly, the lignin-based material, “OGL”, representing the most innovative and sustainability apt option, offered relevant performance only in selected cases. The significant differences in performance among the resins underscore the impact of structural and compositional factors on adsorption efficiency. This study offers valuable insights for investigating and selecting new sustainable materials for treating contaminated water, opening new pathways for targeted and optimized solutions in environmental remediation.
Full article
(This article belongs to the Special Issue Separation Technology for Metal Extraction and Removal)
►▼
Show Figures
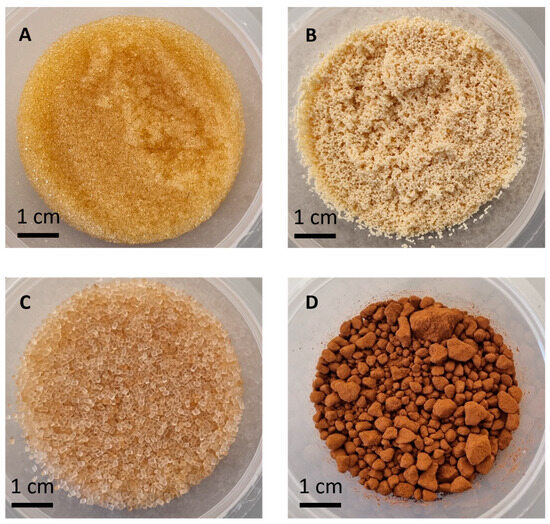
Figure 1
Figure 1
<p>Photographic images of the ion exchange materials utilized: (<b>A</b>) polystyrene–divinylbenzfene resin (PS–DVB), (<b>B</b>) polyacrylic–divinylbenzene resin (PA–DVB), (<b>D</b>) regenerated Nafion granules (TFSA), and (<b>D</b>) lignin-based powder material (OGL).</p> Full article ">Figure 2
<p>Time-dependent concentration of the available metal ions (Cu<sup>2+</sup>, Pb<sup>2+</sup>, Co<sup>2+</sup> and Ni<sup>2+</sup>) in bulk solution (ppm) examined by ICP-EOS. Each panel shows the remaining concentration of a specific metal ion over time (0, 5, 10, 20, and 40 min): (<b>A</b>) Cu<sup>2+</sup> concentration in bulk, (<b>B</b>) Pb<sup>2+</sup> concentration in bulk, (<b>C</b>) Co<sup>2+</sup> concentration in bulk, and (<b>D</b>) Ni<sup>2+</sup> concentration in bulk.</p> Full article ">
<p>Photographic images of the ion exchange materials utilized: (<b>A</b>) polystyrene–divinylbenzfene resin (PS–DVB), (<b>B</b>) polyacrylic–divinylbenzene resin (PA–DVB), (<b>D</b>) regenerated Nafion granules (TFSA), and (<b>D</b>) lignin-based powder material (OGL).</p> Full article ">Figure 2
<p>Time-dependent concentration of the available metal ions (Cu<sup>2+</sup>, Pb<sup>2+</sup>, Co<sup>2+</sup> and Ni<sup>2+</sup>) in bulk solution (ppm) examined by ICP-EOS. Each panel shows the remaining concentration of a specific metal ion over time (0, 5, 10, 20, and 40 min): (<b>A</b>) Cu<sup>2+</sup> concentration in bulk, (<b>B</b>) Pb<sup>2+</sup> concentration in bulk, (<b>C</b>) Co<sup>2+</sup> concentration in bulk, and (<b>D</b>) Ni<sup>2+</sup> concentration in bulk.</p> Full article ">
Open AccessArticle
Activating Peroxymonosulfate by a NovelFe-MOF-C/N Catalyst to Effectively Degrade Sulfamethoxazole: Catalyst Performance and Its Mechanism
by
Zhaowei Wu, Meiyi Hu, Pengfan Zhuo, Jie Wang and Shaoping Tong
Separations 2024, 11(12), 356; https://doi.org/10.3390/separations11120356 - 21 Dec 2024
Abstract
►▼
Show Figures
A composite Fe-MOF-C/N was prepared with Fe-MOF and g-C3N4 precursors, and its activation property of peroxymonosulfate (PMS) in the degradation of Sulfamethoxazole (SMX) was studied. XRD, SEM, and TEM analyses showed that Fe-MOF-C/N was porous and mainly composed of phases
[...] Read more.
A composite Fe-MOF-C/N was prepared with Fe-MOF and g-C3N4 precursors, and its activation property of peroxymonosulfate (PMS) in the degradation of Sulfamethoxazole (SMX) was studied. XRD, SEM, and TEM analyses showed that Fe-MOF-C/N was porous and mainly composed of phases of Fe3N, C0.08Fe1.92, and graphite. XPS indicated that Fe-MOF-C/N mainly had Fe, C, N, and O elements, among which Fe existed in the form of Fe2+ and Fe3+. Fe-MOF-C/N was used to activate PMS to degrade SMX, and the result showed that Fe-MOF-C/N had excellent catalytic performance and 84.17% of SMX could be removed in 8 min. Free radical quenching experiments showed that 1O2 was the main active species in Fe-MOF-C/N/PMS. The stability experiment showed that Fe-MOF-C/N had good stability, and the degradation rate of SMX only decreased by 5.8% after five times of use.
Full article
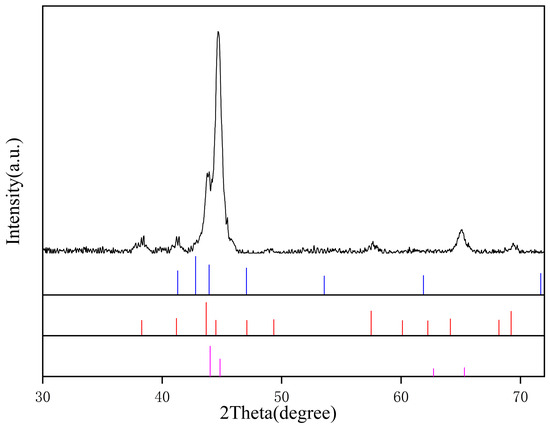
Figure 1
Figure 1
<p>XRD spectra of Fe-MOF-C/N-1:0.5.</p> Full article ">Figure 2
<p>SEM of Fe-MOF-C/N-1:0.5 before (<b>a</b>) and after calcination (<b>b</b>).</p> Full article ">Figure 3
<p>Mapping of Fe-MOF-C/N-1:0.5 after calcination (<b>a</b>); distribution of each element (<b>b</b>); distribution of C (<b>c</b>); distribution of N (<b>d</b>) and distribution of Fe (<b>e</b>).</p> Full article ">Figure 4
<p>TEM image at 100 nm (<b>a,b</b>); TEM image with lattice spacing at 5 nm (<b>c</b>); TEM image with lattice spacing at 10 nm (<b>d</b>) of Fe-MOF-C/N-1:0.5.</p> Full article ">Figure 5
<p>N<sub>2</sub> Absorption/desorption isotherms (<b>a</b>) and pore size distribution (<b>b</b>) of Fe-MOF-C/N-1:0.5.</p> Full article ">Figure 6
<p>XPS spectra of Fe-MOF-C/N-1:0.5 (full spectrum (<b>a</b>) and high-resolution spectra of elements ((<b>b</b>) C, (<b>c</b>) Fe, and (<b>d</b>) N)).</p> Full article ">Figure 7
<p>Efficiencies of Fe-MOF-C/N-1:0.5/PMS in degradation of SMX. Reaction conditions: SMX solution (200 mL 50 mg/L); PMS and Fe-MOF-C/N (0.20 g/L); pH = 7.</p> Full article ">Figure 8
<p>Effect of mass ratio of precursors on degradation efficiency of SMX. Reaction conditions: SMX solution (200 mL 50 mg/L); PMS and Fe-MOF-C/N (0.20 g/L); pH = 7.</p> Full article ">Figure 9
<p>Effect of calcination temperature on degradation of SMX. Reaction conditions: SMX solution (200 mL 50 mg/L); PMS and Fe-MOF-C/N (0.20 g/L); pH = 7.</p> Full article ">Figure 10
<p>XRD spectra of Fe-MOF-C/N-1:0.5 prepared at different calcination temperatures.</p> Full article ">Figure 11
<p>Effect of ethanol, TBA, and FFA on degradation of SMX. Reaction conditions: SMX solution (200 mL 50 mg/L); PMS and Fe-MOF-C/N (0.20 g/L); pH = 7.</p> Full article ">Figure 12
<p>Effect of different initial pH values on degradation efficiency of SMX. Reaction conditions: SMX solution (200 mL 50 mg/L); PMS and Fe-MOF-C/N (0.20 g/L); pH = 7.</p> Full article ">Figure 13
<p>Effect of different dosage of PMS on degradation efficiency of SMX. Reaction conditions: SMX solution (200 mL 50 mg/L) and Fe-MOF-C/N (0.20 g/L); pH = 7.</p> Full article ">Figure 14
<p>Effect of different dosage of Fe-MOF-C/N-1:0.5 on degradation of SMX. Reaction conditions: SMX solution (200 mL 50 mg/L) and PMS (0.20 g/L).</p> Full article ">Figure 15
<p>The adsorption effect of different Fe-MOF-C/N-1:0.5 dosages on SMX. Reaction conditions: SMX solution (200 mL 50 mg/L) and PMS (0.20 g/L).</p> Full article ">Figure 16
<p>Recycling test of Fe-MOF-C/N-1:0.5 for activating PMS. Reaction conditions: SMX solution (200 mL 50 mg/L); PMS and Fe-MOF-C/N (0.20 g/L).</p> Full article ">Figure 17
<p>XRD of fresh and used catalysts.</p> Full article ">Figure 18
<p>Possible degradation pathway of SMX.</p> Full article ">Figure 19
<p>Toxicity analysis of intermediate products of SMX, Lethal concentrations of intermediates products to large dophnia (<b>a</b>); Lethal concentrations of intermediates to fat-heat fish (<b>b</b>); Developmental toxicity (<b>c</b>); Mutagenicity (<b>d</b>).</p> Full article ">
<p>XRD spectra of Fe-MOF-C/N-1:0.5.</p> Full article ">Figure 2
<p>SEM of Fe-MOF-C/N-1:0.5 before (<b>a</b>) and after calcination (<b>b</b>).</p> Full article ">Figure 3
<p>Mapping of Fe-MOF-C/N-1:0.5 after calcination (<b>a</b>); distribution of each element (<b>b</b>); distribution of C (<b>c</b>); distribution of N (<b>d</b>) and distribution of Fe (<b>e</b>).</p> Full article ">Figure 4
<p>TEM image at 100 nm (<b>a,b</b>); TEM image with lattice spacing at 5 nm (<b>c</b>); TEM image with lattice spacing at 10 nm (<b>d</b>) of Fe-MOF-C/N-1:0.5.</p> Full article ">Figure 5
<p>N<sub>2</sub> Absorption/desorption isotherms (<b>a</b>) and pore size distribution (<b>b</b>) of Fe-MOF-C/N-1:0.5.</p> Full article ">Figure 6
<p>XPS spectra of Fe-MOF-C/N-1:0.5 (full spectrum (<b>a</b>) and high-resolution spectra of elements ((<b>b</b>) C, (<b>c</b>) Fe, and (<b>d</b>) N)).</p> Full article ">Figure 7
<p>Efficiencies of Fe-MOF-C/N-1:0.5/PMS in degradation of SMX. Reaction conditions: SMX solution (200 mL 50 mg/L); PMS and Fe-MOF-C/N (0.20 g/L); pH = 7.</p> Full article ">Figure 8
<p>Effect of mass ratio of precursors on degradation efficiency of SMX. Reaction conditions: SMX solution (200 mL 50 mg/L); PMS and Fe-MOF-C/N (0.20 g/L); pH = 7.</p> Full article ">Figure 9
<p>Effect of calcination temperature on degradation of SMX. Reaction conditions: SMX solution (200 mL 50 mg/L); PMS and Fe-MOF-C/N (0.20 g/L); pH = 7.</p> Full article ">Figure 10
<p>XRD spectra of Fe-MOF-C/N-1:0.5 prepared at different calcination temperatures.</p> Full article ">Figure 11
<p>Effect of ethanol, TBA, and FFA on degradation of SMX. Reaction conditions: SMX solution (200 mL 50 mg/L); PMS and Fe-MOF-C/N (0.20 g/L); pH = 7.</p> Full article ">Figure 12
<p>Effect of different initial pH values on degradation efficiency of SMX. Reaction conditions: SMX solution (200 mL 50 mg/L); PMS and Fe-MOF-C/N (0.20 g/L); pH = 7.</p> Full article ">Figure 13
<p>Effect of different dosage of PMS on degradation efficiency of SMX. Reaction conditions: SMX solution (200 mL 50 mg/L) and Fe-MOF-C/N (0.20 g/L); pH = 7.</p> Full article ">Figure 14
<p>Effect of different dosage of Fe-MOF-C/N-1:0.5 on degradation of SMX. Reaction conditions: SMX solution (200 mL 50 mg/L) and PMS (0.20 g/L).</p> Full article ">Figure 15
<p>The adsorption effect of different Fe-MOF-C/N-1:0.5 dosages on SMX. Reaction conditions: SMX solution (200 mL 50 mg/L) and PMS (0.20 g/L).</p> Full article ">Figure 16
<p>Recycling test of Fe-MOF-C/N-1:0.5 for activating PMS. Reaction conditions: SMX solution (200 mL 50 mg/L); PMS and Fe-MOF-C/N (0.20 g/L).</p> Full article ">Figure 17
<p>XRD of fresh and used catalysts.</p> Full article ">Figure 18
<p>Possible degradation pathway of SMX.</p> Full article ">Figure 19
<p>Toxicity analysis of intermediate products of SMX, Lethal concentrations of intermediates products to large dophnia (<b>a</b>); Lethal concentrations of intermediates to fat-heat fish (<b>b</b>); Developmental toxicity (<b>c</b>); Mutagenicity (<b>d</b>).</p> Full article ">
Open AccessArticle
Evaluating Bioflocculation Harvesting of Freshwater and Marine Microalgae Using Exopolysaccharides (EPSs) from Klebsiella sp.
by
Yicheng Yuan, Jingxuan Lu and Quan Wang
Separations 2024, 11(12), 355; https://doi.org/10.3390/separations11120355 - 19 Dec 2024
Abstract
The rising global energy demand and environmental concerns associated with fossil fuels have intensified interest in sustainable biofuel sources, with microalgae emerging as a viable candidate due to its high biomass yield and efficient CO2 conversion. However, the economic feasibility of microalgal
[...] Read more.
The rising global energy demand and environmental concerns associated with fossil fuels have intensified interest in sustainable biofuel sources, with microalgae emerging as a viable candidate due to its high biomass yield and efficient CO2 conversion. However, the economic feasibility of microalgal biofuels is currently challenged by costly harvesting processes. This study investigates the use of exopolysaccharides (EPSs) derived from Klebsiella sp. as an environmentally friendly bioflocculant for harvesting two microalgae species: Raphidocelis subcapitata and Dunaliella salina. Comparative flocculation experiments revealed that Klebsiella EPS promotes efficient aggregation in R. subcapitata, achieving over 90% flocculation efficiency, while performance with D. salina was impacted by high salinity, which reduced charge neutralization and bridging effects. Structural analyses using FTIR, 3D-EEM, CLSM, and XPS elucidated the EPS composition, underscoring the roles of polysaccharides and proteins in facilitating microalgal aggregation. The findings indicate that Klebsiella EPS offers a sustainable alternative to chemical flocculants, supporting eco-friendly biofuel production and potential applications in wastewater treatment. This approach provides insights into optimizing EPS-based flocculation for diverse environmental conditions, paving the way for more sustainable biomass recovery practices.
Full article
(This article belongs to the Special Issue Separation Technology for Solid Waste Treatment and Recycling)
►▼
Show Figures
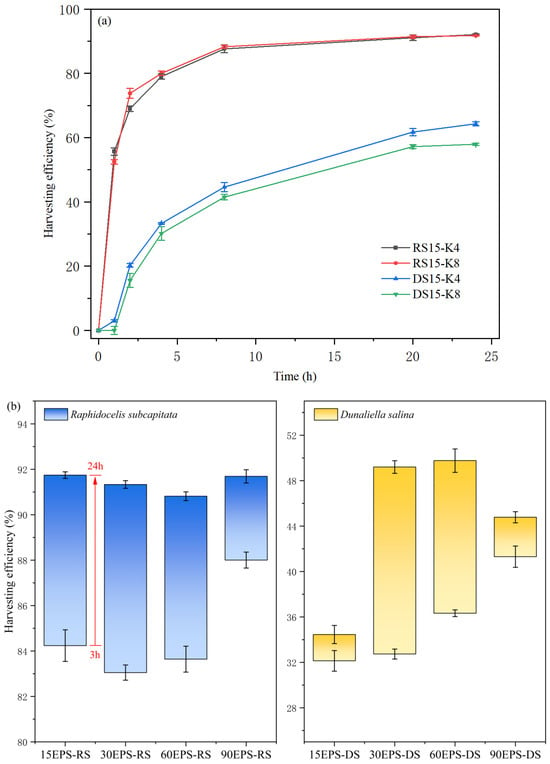
Figure 1
Figure 1
<p>Harvesting efficiency of (<b>a</b>) directly added <span class="html-italic">Klebsiella</span> sp. suspensions, (<b>b</b>) EPS extracted from <span class="html-italic">Klebsiella</span> sp. on two microalgae species.</p> Full article ">Figure 2
<p>Floc structures of (<b>a</b>) RS15-K4, (<b>b</b>) RS15-K8, (<b>c</b>) 90EPS-RS, (<b>d</b>) DS15-K4, (<b>e</b>) DS15-K8, and (<b>f</b>) 90EPS-DS under 20× optical microscopy.</p> Full article ">Figure 3
<p>SEM imaging of floc structures: (<b>a</b>) RS15-K4, (<b>b</b>) RS15-K8, (<b>c</b>) 90EPS-RS, (<b>d</b>) DS15-K4, (<b>e</b>) DS15-K8, and (<b>f</b>) 90EPS-DS.</p> Full article ">Figure 4
<p>Particle size distributions of floc structures: (<b>a</b>) <span class="html-italic">R. subcapitata</span>, (<b>b</b>) <span class="html-italic">D. salina</span>, and (<b>c</b>) zeta-potential change after the addition of <span class="html-italic">Klebsiella</span> sp. broth.</p> Full article ">Figure 5
<p>(<b>a</b>) FTIR spectra detailing the chemical composition of EPS. (<b>b1</b>–<b>b3</b>) The 3D-EEM spectra for S-EPS, LB-EPS, and TB-EPS. (<b>c1</b>–<b>c5</b>) CLSM images of <span class="html-italic">Klebsiella</span>: general cellular staining (<b>c1</b>), specific red staining for lipids (<b>c2</b>), blue staining for proteins (<b>c3</b>), green staining for polysaccharides (<b>c4</b>), and a composite overlay of all stains (<b>c5</b>). (<b>d</b>) Pie chart showing the composition ratios of S-EPS, LB-EPS, and TB-EPS in EPS.</p> Full article ">Figure 6
<p>XPS spectra for total EPS and S-EPS: (<b>a1</b>,<b>b1</b>,<b>c1</b>) represent the C1s, O1s, and N1s spectra for total EPS, respectively, while (<b>a2</b>,<b>b2</b>,<b>c2</b>) show the high-resolution C1s, O1s, and N1s spectra for S-EPS.</p> Full article ">
<p>Harvesting efficiency of (<b>a</b>) directly added <span class="html-italic">Klebsiella</span> sp. suspensions, (<b>b</b>) EPS extracted from <span class="html-italic">Klebsiella</span> sp. on two microalgae species.</p> Full article ">Figure 2
<p>Floc structures of (<b>a</b>) RS15-K4, (<b>b</b>) RS15-K8, (<b>c</b>) 90EPS-RS, (<b>d</b>) DS15-K4, (<b>e</b>) DS15-K8, and (<b>f</b>) 90EPS-DS under 20× optical microscopy.</p> Full article ">Figure 3
<p>SEM imaging of floc structures: (<b>a</b>) RS15-K4, (<b>b</b>) RS15-K8, (<b>c</b>) 90EPS-RS, (<b>d</b>) DS15-K4, (<b>e</b>) DS15-K8, and (<b>f</b>) 90EPS-DS.</p> Full article ">Figure 4
<p>Particle size distributions of floc structures: (<b>a</b>) <span class="html-italic">R. subcapitata</span>, (<b>b</b>) <span class="html-italic">D. salina</span>, and (<b>c</b>) zeta-potential change after the addition of <span class="html-italic">Klebsiella</span> sp. broth.</p> Full article ">Figure 5
<p>(<b>a</b>) FTIR spectra detailing the chemical composition of EPS. (<b>b1</b>–<b>b3</b>) The 3D-EEM spectra for S-EPS, LB-EPS, and TB-EPS. (<b>c1</b>–<b>c5</b>) CLSM images of <span class="html-italic">Klebsiella</span>: general cellular staining (<b>c1</b>), specific red staining for lipids (<b>c2</b>), blue staining for proteins (<b>c3</b>), green staining for polysaccharides (<b>c4</b>), and a composite overlay of all stains (<b>c5</b>). (<b>d</b>) Pie chart showing the composition ratios of S-EPS, LB-EPS, and TB-EPS in EPS.</p> Full article ">Figure 6
<p>XPS spectra for total EPS and S-EPS: (<b>a1</b>,<b>b1</b>,<b>c1</b>) represent the C1s, O1s, and N1s spectra for total EPS, respectively, while (<b>a2</b>,<b>b2</b>,<b>c2</b>) show the high-resolution C1s, O1s, and N1s spectra for S-EPS.</p> Full article ">
Open AccessArticle
Optimization of Extraction of Luteolin from Schisandra chinensis by Ionic Liquid–Enzyme Complex System and Antioxidant Study Analysis
by
Jingwei Hao, Nan Dong, Yifan Sun, Xiaoxia Lu, Yingying Pei, Yi Zhou, Xiangkun Zhou and Heming Liu
Separations 2024, 11(12), 354; https://doi.org/10.3390/separations11120354 - 19 Dec 2024
Abstract
►▼
Show Figures
The luteolin in Schisandra chinensis [Schisandraceae Schisandra (Turcz.) Baill.] were extracted by ultrasonic extraction assisted by an ionic liquid–enzyme composite system, and the content of luteolins was determined using high-performance liquid chromatography (HPLC). This process was initially conducted through a one-factor experiment and
[...] Read more.
The luteolin in Schisandra chinensis [Schisandraceae Schisandra (Turcz.) Baill.] were extracted by ultrasonic extraction assisted by an ionic liquid–enzyme composite system, and the content of luteolins was determined using high-performance liquid chromatography (HPLC). This process was initially conducted through a one-factor experiment and a Box–Behnken combinatorial design of response surface method. The extraction process was optimized, and the results demonstrated that the optimal extraction conditions were 13.31% enzyme addition, 0.53 mol/L ionic liquid concentration, 173.47 min ultrasonic shaking, and 0.2266 mg/g, which was 4.88 times higher than that of the traditional reflux extraction. Secondly, the antioxidant function of luteolins was studied based on network pharmacology. For the study of the antioxidant mechanism of luteolin, the herb group identification database, SwissTargetPrediction on luteolins target prediction, and GeneCards database to achieve the antioxidant target were used. For the analysis of the intersection of the target protein interactions, GO bioanalysis and KEGG signaling pathway enrichment analysis were used. There were 57 overlapping targets of luteolin and antioxidants, including AKT1, MMP9, ESR1, EGFR, and SRC. GO function and KEGG pathway enrichment analysis showed that luteolin antioxidants were related to zoerythromycin metabolic process, adriamycin metabolic process, negative regulation of apoptotic process, endocrine resistance and oxidoreductase. The key targets in the pathways, such as luteolin AKT1 and MMP9, exert antioxidant effects. The antioxidant activity of luteolins was investigated by determining the scavenging ability of luteolins against two types of free radicals: 2,2-bipyridine-bis(3-ethyl-benzothiazole-6-sulfonic acid) diammonium salt (ABTS+) free radicals and 1,1-diphenyl-2-trinitrophenylhydrazine free radicals (DPPH-). The results of the antioxidant test demonstrated that the ABTS radical scavenging rate was 87.26%, and the DPPH radical scavenging rate was 93.85% when the quality concentration of Schisandra luteolins was 0.1 mg/g, indicating the potential of this natural antioxidant. This method of extracting Schisandra chinensis luteolins is highly productive, environmentally friendly, and practical, and it facilitates the development and utilization of industrial Schisandra chinensis.
Full article
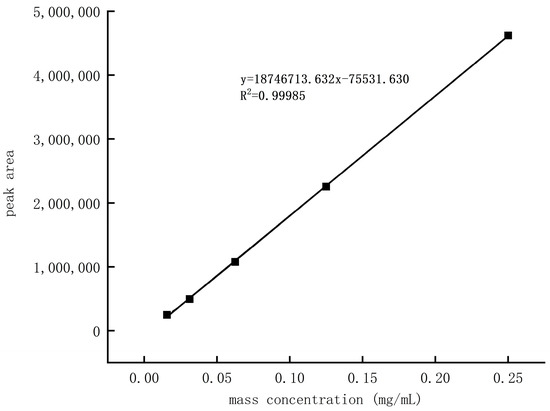
Figure 1
Figure 1
<p>Standard curve of luteolin.</p> Full article ">Figure 2
<p>The effect of ionic liquid type on luteolin yield (<b>a</b>); the effect of ionic liquid concentration on luteolin yield (<b>b</b>); the effect of enzyme addition amount on luteolin yield (<b>c</b>); the effect of solid–liquid ratio on luteolin yield (<b>d</b>), the effect of ultrasonic time on luteolin yield (<b>e</b>); and the effect of enzyme addition amount on luteolin yield. Effect of ultrasonic extraction temperature on luteolin yield (<b>f</b>); effect of enzymolysis time on luteolin yield (<b>g</b>); effect of enzymolysis temperature on luteolin yield (<b>h</b>). Different letters labeled in the bar graph indicate a significant difference (<span class="html-italic">p</span> < 0.05) and the same letter indicates a non-significant difference (<span class="html-italic">p</span> > 0.05).</p> Full article ">Figure 2 Cont.
<p>The effect of ionic liquid type on luteolin yield (<b>a</b>); the effect of ionic liquid concentration on luteolin yield (<b>b</b>); the effect of enzyme addition amount on luteolin yield (<b>c</b>); the effect of solid–liquid ratio on luteolin yield (<b>d</b>), the effect of ultrasonic time on luteolin yield (<b>e</b>); and the effect of enzyme addition amount on luteolin yield. Effect of ultrasonic extraction temperature on luteolin yield (<b>f</b>); effect of enzymolysis time on luteolin yield (<b>g</b>); effect of enzymolysis temperature on luteolin yield (<b>h</b>). Different letters labeled in the bar graph indicate a significant difference (<span class="html-italic">p</span> < 0.05) and the same letter indicates a non-significant difference (<span class="html-italic">p</span> > 0.05).</p> Full article ">Figure 3
<p>Response surface contours and curves between each factor and yield.</p> Full article ">Figure 3 Cont.
<p>Response surface contours and curves between each factor and yield.</p> Full article ">Figure 4
<p>Interactive target information of luteolin and antioxidant.</p> Full article ">Figure 5
<p>Drug—component—gene target network of luteolin antioxidant. The red triangle represents schisandra, the yellow triangle represents luteolin, and the blue rectangle represents the intersection target.</p> Full article ">Figure 6
<p>PPI network of luteolin antioxidant function.</p> Full article ">Figure 7
<p>Top 10 core targets in the PPI network of luteolin antioxidant function. The ranking of core targets is inversely proportional to the darkness of the color.</p> Full article ">Figure 8
<p>Bubble diagram of GO function and KEGG path enrichment analysis. (<b>A</b>): BP; (<b>B</b>): MF; (<b>C</b>): CC; (<b>D</b>): KEGG.</p> Full article ">Figure 8 Cont.
<p>Bubble diagram of GO function and KEGG path enrichment analysis. (<b>A</b>): BP; (<b>B</b>): MF; (<b>C</b>): CC; (<b>D</b>): KEGG.</p> Full article ">Figure 9
<p>Scavenging ability of luteolin on free radicals. Different letters labeled in the bar graph indicate a significant difference (<span class="html-italic">p</span> < 0.05) and the same letter indicates a non-significant difference (<span class="html-italic">p</span> > 0.05).</p> Full article ">
<p>Standard curve of luteolin.</p> Full article ">Figure 2
<p>The effect of ionic liquid type on luteolin yield (<b>a</b>); the effect of ionic liquid concentration on luteolin yield (<b>b</b>); the effect of enzyme addition amount on luteolin yield (<b>c</b>); the effect of solid–liquid ratio on luteolin yield (<b>d</b>), the effect of ultrasonic time on luteolin yield (<b>e</b>); and the effect of enzyme addition amount on luteolin yield. Effect of ultrasonic extraction temperature on luteolin yield (<b>f</b>); effect of enzymolysis time on luteolin yield (<b>g</b>); effect of enzymolysis temperature on luteolin yield (<b>h</b>). Different letters labeled in the bar graph indicate a significant difference (<span class="html-italic">p</span> < 0.05) and the same letter indicates a non-significant difference (<span class="html-italic">p</span> > 0.05).</p> Full article ">Figure 2 Cont.
<p>The effect of ionic liquid type on luteolin yield (<b>a</b>); the effect of ionic liquid concentration on luteolin yield (<b>b</b>); the effect of enzyme addition amount on luteolin yield (<b>c</b>); the effect of solid–liquid ratio on luteolin yield (<b>d</b>), the effect of ultrasonic time on luteolin yield (<b>e</b>); and the effect of enzyme addition amount on luteolin yield. Effect of ultrasonic extraction temperature on luteolin yield (<b>f</b>); effect of enzymolysis time on luteolin yield (<b>g</b>); effect of enzymolysis temperature on luteolin yield (<b>h</b>). Different letters labeled in the bar graph indicate a significant difference (<span class="html-italic">p</span> < 0.05) and the same letter indicates a non-significant difference (<span class="html-italic">p</span> > 0.05).</p> Full article ">Figure 3
<p>Response surface contours and curves between each factor and yield.</p> Full article ">Figure 3 Cont.
<p>Response surface contours and curves between each factor and yield.</p> Full article ">Figure 4
<p>Interactive target information of luteolin and antioxidant.</p> Full article ">Figure 5
<p>Drug—component—gene target network of luteolin antioxidant. The red triangle represents schisandra, the yellow triangle represents luteolin, and the blue rectangle represents the intersection target.</p> Full article ">Figure 6
<p>PPI network of luteolin antioxidant function.</p> Full article ">Figure 7
<p>Top 10 core targets in the PPI network of luteolin antioxidant function. The ranking of core targets is inversely proportional to the darkness of the color.</p> Full article ">Figure 8
<p>Bubble diagram of GO function and KEGG path enrichment analysis. (<b>A</b>): BP; (<b>B</b>): MF; (<b>C</b>): CC; (<b>D</b>): KEGG.</p> Full article ">Figure 8 Cont.
<p>Bubble diagram of GO function and KEGG path enrichment analysis. (<b>A</b>): BP; (<b>B</b>): MF; (<b>C</b>): CC; (<b>D</b>): KEGG.</p> Full article ">Figure 9
<p>Scavenging ability of luteolin on free radicals. Different letters labeled in the bar graph indicate a significant difference (<span class="html-italic">p</span> < 0.05) and the same letter indicates a non-significant difference (<span class="html-italic">p</span> > 0.05).</p> Full article ">
Open AccessReview
The Removal of Organic Pollutants and Ammonia Nitrogen from High-Salt Wastewater by the Electro-Chlorination Process and Its Mechanism
by
Yujun Zhou, Tangrui Hou and Bo Zhou
Separations 2024, 11(12), 353; https://doi.org/10.3390/separations11120353 - 18 Dec 2024
Abstract
Electro-chlorination (E-Cl) is an emerging and promising electrochemical advanced oxidation technology for wastewater treatment with the advantages of high efficiency, deep mineralization, a green process, and easy operation. It was found that the mechanism of pollutant removal by electro-chlorination mainly involves an indirect
[...] Read more.
Electro-chlorination (E-Cl) is an emerging and promising electrochemical advanced oxidation technology for wastewater treatment with the advantages of high efficiency, deep mineralization, a green process, and easy operation. It was found that the mechanism of pollutant removal by electro-chlorination mainly involves an indirect oxidation process, in which pollutant removal is mainly driven by the intermediate active species, especially RCS and chlorine radicals, with a strong oxidization ability produced at the anodes. In this work, we summarized the principles and pathways of the removal/degradation of pollutants (organic pollutants and ammonia nitrogen) by E-Cl and the major affecting factors including the applied current density, voltage, electrolyte concentration, initial pH value, etc. In the E-Cl system, the DSA and BDD electrodes were the most widely used electrode materials. The flow-through electrode reactor was considered to be the most promising reactor since it had a high porosity and large pore size, which could effectively improve the mass transfer efficiency and electron transfer efficiency of the reaction. Of the many detection methods for chlorine radicals and RCS, electron paramagnetic resonance (EPR) and spectrophotometry with N, N-diethyl-1,4-phenylenediamine sulfate (DPD) as the chromogenic agent were the two most widely used methods. Overall, the E-Cl process had excellent performance and prospects in treating salt-containing wastewater.
Full article
(This article belongs to the Special Issue Design and Preparation of Environmental Functional Materials for Pollutant Removal)
►▼
Show Figures
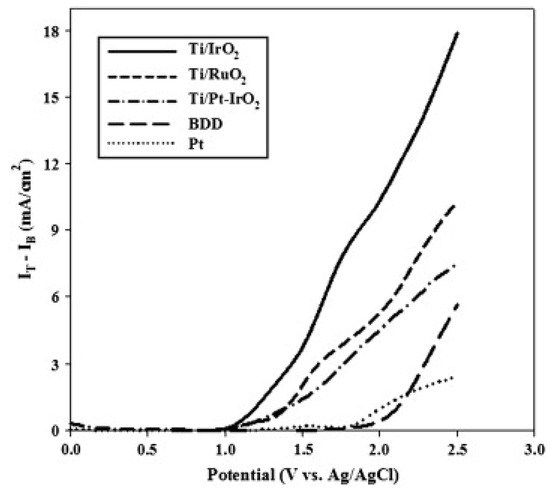
Figure 1
Figure 1
<p>Comparison of voltammetric currents associated with the evolution of chlorine for five electrode materials [<a href="#B49-separations-11-00353" class="html-bibr">49</a>].</p> Full article ">Figure 2
<p>Configuration of flow-through reactor [<a href="#B53-separations-11-00353" class="html-bibr">53</a>].</p> Full article ">Figure 3
<p>Mechanism of ECl process for ammonia nitrogen removal [<a href="#B14-separations-11-00353" class="html-bibr">14</a>].</p> Full article ">Figure 4
<p>Mechanism of E-Cl process for ofloxacin removal [<a href="#B76-separations-11-00353" class="html-bibr">76</a>].</p> Full article ">Figure 5
<p>EPR scan result of DMPO-X [<a href="#B28-separations-11-00353" class="html-bibr">28</a>].</p> Full article ">Figure 6
<p>Quenching experiments for RCS determination in E-Cl system. Na<sub>2</sub>S<sub>2</sub>O<sub>3</sub> = MeOH = TBA = 50 mmol/L, NB = 1000 mg/L [<a href="#B10-separations-11-00353" class="html-bibr">10</a>].</p> Full article ">Figure 7
<p>Ammonia nitrogen loading efficiency and removal efficiency (<b>a</b>), Nitrite concentration of influent and removal/accumulation of nitrite (<b>b</b>) in MEBR and MBR [<a href="#B101-separations-11-00353" class="html-bibr">101</a>].</p> Full article ">
<p>Comparison of voltammetric currents associated with the evolution of chlorine for five electrode materials [<a href="#B49-separations-11-00353" class="html-bibr">49</a>].</p> Full article ">Figure 2
<p>Configuration of flow-through reactor [<a href="#B53-separations-11-00353" class="html-bibr">53</a>].</p> Full article ">Figure 3
<p>Mechanism of ECl process for ammonia nitrogen removal [<a href="#B14-separations-11-00353" class="html-bibr">14</a>].</p> Full article ">Figure 4
<p>Mechanism of E-Cl process for ofloxacin removal [<a href="#B76-separations-11-00353" class="html-bibr">76</a>].</p> Full article ">Figure 5
<p>EPR scan result of DMPO-X [<a href="#B28-separations-11-00353" class="html-bibr">28</a>].</p> Full article ">Figure 6
<p>Quenching experiments for RCS determination in E-Cl system. Na<sub>2</sub>S<sub>2</sub>O<sub>3</sub> = MeOH = TBA = 50 mmol/L, NB = 1000 mg/L [<a href="#B10-separations-11-00353" class="html-bibr">10</a>].</p> Full article ">Figure 7
<p>Ammonia nitrogen loading efficiency and removal efficiency (<b>a</b>), Nitrite concentration of influent and removal/accumulation of nitrite (<b>b</b>) in MEBR and MBR [<a href="#B101-separations-11-00353" class="html-bibr">101</a>].</p> Full article ">
Open AccessArticle
Volatile Organic Compounds in Honey: Tandem Mass Spectrometry as Tool to Quantitate Priority VOCs
by
Evangelia N. Tzanetou, Efstathia Vousaxaki, Kyriaki Machera, Jozef van der Steen and Konstantinos M. Kasiotis
Separations 2024, 11(12), 352; https://doi.org/10.3390/separations11120352 - 17 Dec 2024
Abstract
A headspace gas chromatographic tandem mass spectrometric (HS-GC-MS/MS) method was developed and fully validated, aiming for the simultaneous determination of 25 volatile organic compounds (VOCs, some of them previously unreported in honey bee studies) in 52 selected honey samples from Greece. The HS
[...] Read more.
A headspace gas chromatographic tandem mass spectrometric (HS-GC-MS/MS) method was developed and fully validated, aiming for the simultaneous determination of 25 volatile organic compounds (VOCs, some of them previously unreported in honey bee studies) in 52 selected honey samples from Greece. The HS conditions were optimized, and method validation criteria were extensively investigated. The existence impact of the matrix effect was assessed, and matrix-matched calibration curves were developed for quantification purposes. The limits of quantification of the 25 analytes ranged from 0.2 ng g−1 to 0.6 ng g−1. Isoprene was the most commonly detected VOC, followed by octane and styrene. Other detected VOCs include benzene, n-hexane, trimethylbenzenes, xylenes, toluene, and p-dichlorobenzene. Concentrations fluctuated from 0.5 ng g−1 for isoprene and toluene, as well as 1,2,4-trimethylbenzene, to 22.6 ng g−1 for isoprene. Despite VOCs not being at the forefront of honey’s potential contamination, their prevalence in honey can provide significant data for human health risk assessment, considering their undisputable widespread consumption and the documented potential toxicity of VOCs in humans. In this sense, risk assessment for adults and children, as depicted in the hazard quotient and index and carcinogenic risk determination, did not disclose any potential threat after consumption of the investigated honey samples.
Full article
(This article belongs to the Special Issue Chemical and Contaminant Residue Analysis via Chromatography)
►▼
Show Figures
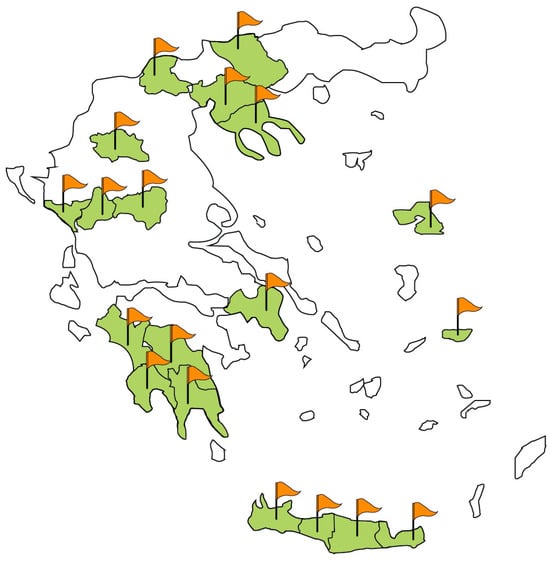
Figure 1
Figure 1
<p>Sample Collection points in Greece.</p> Full article ">Figure 2
<p>Effect of incubation time on the response of VOCs on mass spectrometer (incubation temperature at 80 °C).</p> Full article ">Figure 3
<p>Effect of incubation temperature on the response of VOCs on mass spectrometer (incubation time at 15 min).</p> Full article ">Figure 4
<p>Effect of matrix modifier on the response of VOCs on mass spectrometer.</p> Full article ">Figure 5
<p>MRM chromatograms of isoprene (<b>a</b>) and styrene (<b>b</b>) in a positive honey sample.</p> Full article ">
<p>Sample Collection points in Greece.</p> Full article ">Figure 2
<p>Effect of incubation time on the response of VOCs on mass spectrometer (incubation temperature at 80 °C).</p> Full article ">Figure 3
<p>Effect of incubation temperature on the response of VOCs on mass spectrometer (incubation time at 15 min).</p> Full article ">Figure 4
<p>Effect of matrix modifier on the response of VOCs on mass spectrometer.</p> Full article ">Figure 5
<p>MRM chromatograms of isoprene (<b>a</b>) and styrene (<b>b</b>) in a positive honey sample.</p> Full article ">
Journal Menu
► ▼ Journal Menu-
- Separations Home
- Aims & Scope
- Editorial Board
- Reviewer Board
- Topical Advisory Panel
- Instructions for Authors
- Special Issues
- Topics
- Sections & Collections
- Article Processing Charge
- Indexing & Archiving
- Editor’s Choice Articles
- Most Cited & Viewed
- Journal Statistics
- Journal History
- Journal Awards
- Society Collaborations
- Editorial Office
Journal Browser
► ▼ Journal BrowserHighly Accessed Articles
Latest Books
E-Mail Alert
News
Topics
Topic in
Beverages, Foods, Molecules, Nutrients, Separations, Chemosensors
Advances in Analysis of Food and Beverages
Topic Editors: Anna M. Kaczmarek, Constantinos G. TsiafoulisDeadline: 31 January 2025
Topic in
Analytica, Metabolites, Molecules, Separations, Toxins
Counter-Current Chromatography in Natural Products Isolation
Topic Editors: Shihua Wu, Zhi Yang, Xinyi HuangDeadline: 31 March 2025
Topic in
Analytica, Foods, Molecules, Processes, Separations
New Trends on Separation and Extraction of Bioactive Compounds and Respective Applications
Topic Editors: Isabel Maria Duque Martins, Madalena M. DiasDeadline: 30 April 2025
Topic in
Analytica, Biomolecules, Metabolites, Molecules, Separations, Spectroscopy Journal
Advances in Separation Methods for Metabolomics and Lipidomics
Topic Editors: Eduardo Sommella, Giulia Mazzoccanti, Emanuela SalviatiDeadline: 31 May 2025
Conferences
Special Issues
Special Issue in
Separations
Analytical Pyrolysis–Gas Chromatography–Mass Spectrometry of Synthetic Polymers and Biopolymers
Guest Editor: Peter KuschDeadline: 20 January 2025
Special Issue in
Separations
Advanced Research on Extraction and Analysis of Plant Extracts
Guest Editor: Lina RaudonėDeadline: 10 February 2025
Special Issue in
Separations
Novel Methods for the Analysis of Active and Toxic Components in Food
Guest Editors: Lorena González-Gómez, Sonia Morante ZarceroDeadline: 10 February 2025
Special Issue in
Separations
Adsorption Process in Chemical Engineering
Guest Editors: Adrian Bonilla-Petriciolet, Didilia Ileana Mendoza-Castillo, Hilda Elizabeth Reynel-ÁvilaDeadline: 10 February 2025
Topical Collections
Topical Collection in
Separations
Synthetic Membrane Separation Science and Technology
Collection Editors: Mohamed Khayet, Elena Guillen Burrieza
Topical Collection in
Separations
Feature Paper Collection in Section Chromatographic Separations
Collection Editors: Victoria Samanidou, Evroula Hapeshi
Topical Collection in
Separations
Review Papers of SeparationsCollection Editors: Frank L. Dorman, Gautam Sethi
Topical Collection in
Separations
Feature Paper Collection in Section 'Environmental Separations'
Collection Editors: Maria Elizabeth Tiritan, Cláudia Maria Rosa Ribeiro